The more the better – determining the optimal range when performing single-vesicle phenotyping
DOI: https://doi.org/10.47184/tev.2021.01.03The characterization of extracellular vesicles (EVs) has evolved rapidly in recent years due to advances in straightforward technologies. Based on these more sensitive methods, it is now possible to describe EV populations in their entirety more precisely. However, these applications require an equivalently delicate experiment design and optimization steps to draw valid conclusions in the end. One of these methods is represented by the highly sensitive nanoflow cytometry (nFCM), by which particles can be analyzed not only on their size (< 40 nm) and concentration but also concerning surface markers. In this work, we addressed some of the potential caveats of this method, especially when characterizing particles with fluorescently labelled antibodies. In particular, we show, when using low particle concentrations, which are inevitably encountered when working with EVs, the characterization of surface markers is prone to significantly varying. We hypothesized that these technical limitations could respond to the stickiness of EVs and should be properly counteracted. As a reference, we strongly recommend performing particle number-based comparisons with at least 109 particles as staining input in nFCM analyses. Moreover, we provided representative particle-number based immunoblotting results, underlying the significance of this parameter as a normalizer in future EV research.
Keywords: Extracellular vesicles, size-exclusion chromatography, single-vesicle fluorescence analysis, standardization
Introduction
Extracellular vesicles (EVs) are omnipresent lipid particles released by all cells and are considered to play an important role in cell-to-cell communication under both physiological as well as pathological conditions. Among others, microvesicles that directly bud from the plasma membrane and exosomes, which originate from the endosomal compartment, represent the two major types of EVs that have attracted the attention of the scientific community in recent years. Although, both EV subtypes can be distinguished by their source of origin in theory, they also share common biophysical and biochemical peculiarities such as size and markers [for review see 1].
To improve the characterization of the various EV subtypes, several technologies have been developed in recent years [2, 3], which allow for the phenotyping of single particles by individually detecting specific surface molecules. On the one hand, this methodology increases reproducibility as on the other hand, it allows a more detailed description of the distinct EVs compared to the early years of EV characterization. Flow cytometry (FC) approaches have been predominantly used to characterize EVs in detail. However, some drawbacks of standard FC for EV analyses include but are not limited to inaccurate sizing estimation, the necessity of suitable instrumentation as well a certain level of expertise [4].
The progression of standard FC to nanoflow range (also referred to as, nano flow cytometry, nFCM) has significantly improved the characterization of EVs in terms of their size by single-particle measurements by circumventing the detection of so-called swarm events, which are often detected in conventional flow cytometry when analysing EVs [3, 5]. EV research and its representatives by the International Society for Extracellular Vesicles (ISEV) have pointed out several times the importance of reproducibility and standardization, especially when novel approaches raised and need to be implemented in different settings [4]. Thus, in this work, we aim to contribute to the description of some important technical issues observed when performing fluorescence analyses by nFCM to reduce any potential bias in future research. In particular, we have demonstrated that the total particle number used as input for the analysis can influence the percentage of positive events detected by nFCM (output) under certain staining conditions, independently of the surface marker analysed.
Finally, in this technical note we have also included a serial dilution of particles for the detection of typical intravesicular markers such as Alix, flotillin-1 (FLOT-1), and TSG101, by SDS-PAGE, in which a limiting particle number showed an equivalent detection level compared to nFCM analyses. Low particle yields are a common limitation in EV research, especially when working with primary material, becoming an important target for confounding and variable results. When EV release is impaired under certain treatments compared to controls, comparative fluorescence nFCM analysis could introduce a technical bias if comparisons are based on volume : volume proceedings instead of total particle number even for cell culture models. In sum, our results have shown that the more particles used as input material, the more reliable results we obtain, indicating an unexpected variable that needs to be critically considered in single-EV fluorescence analyses and to be implemented in the field for the upcoming years.
Results and discussion
Purification and initial characterization of ES-2 EVs
For validating the performance of nFCM, we isolated EVs from the ovarian clear-cell carcinoma cell line ES-2. We specifically selected this cell line because, according to our experience, we achieve relatively high quantities of EVs on which, in addition, the classic EV markers CD9, CD63 and CD81 can be detected quite efficiently using (nano) FC. EVs were isolated using a combination of ultra/centrifugation and size-exclusion chromatography (SEC) (Figure 1A).
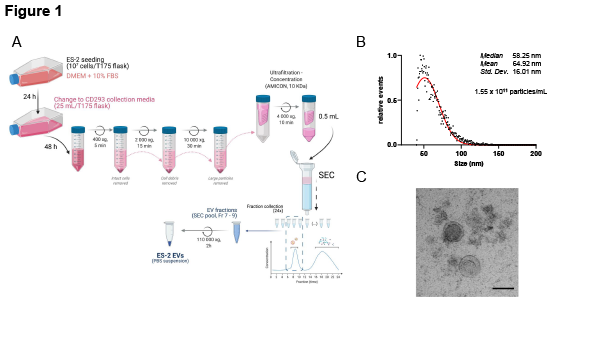
Figure 1: Characterization of ES-2 EV samples. (A) Schematic overview of EV purification from cell culture supernatant. EVs were purified by a combination of differential centrifugation steps, followed by ultrafiltration and size-exclusion chromatography (SEC). The EV containing fractions were pooled and subjected to a final ultracentrifugation step. Subsequently, the EVs were resuspended in freshly filtered PBS (0.1 μm ø) and kept at -20 °C for further analyses. A total of 6 x T175 flasks were used as input in this experiment. (B) Nano flow cytometry (nFCM) analysis depicting the size distribution (diameter of particles, nm) and concentration of particles. Detected vesicles ranged from 40 to 200 nm and bin size was set at 0.5 nm. (C) Electron microscopy of purified vesicles. Scale bar, 100 nm.
In brief, we isolated EVs from cell culture supernatant by differential centrifugation to concentrate extracellular particles. Subsequently, we subjected our enriched particles to SEC to separate our vesicles from co-precipitated proteins and to remove potentially remaining polymers. After a final ultracentrifugation step to concentrate our vesicles, we assessed the specific enrichment of EVs by using nFCM (based on standard beads) (Figure 1B) and transmission electron microscopy (TEM) (Figure 1C). Size and concentration measurements by nFCM resulted in a relatively homogeneous distribution of detected particles with a median diameter of 58.25 +/- 16.01 nm and a concentration of 1.55 x 1011 particles/mL (Figure 1B). Isolated vesicles showed the characteristic cup-shaped morphology reported for EVs in common (Figure 1C). Although cup-shaping is mainly driven by dehydration during sample preparation for TEM, it can be used as a kind of quality control for analyzing lipid-bilayer vesicles [6].
The number of stained EVs affects the detection rate of positive events in single-vesicle fluorescence analyses
Since other approaches for particles analysis revealed that the total number of diluted vesicles do not always reflected the dilution factor correctly [7], we first determined the performance of the NanoAnalyzer by measuring a serial dilution of purified EVs (Figure 2A).
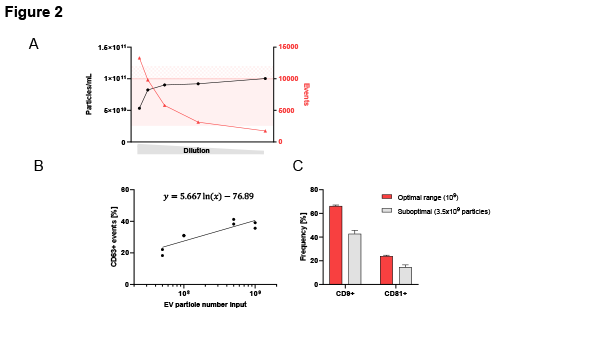
Figure 2: EV particle number negatively impacts the detection of positive events when working under sub-optimal conditions. (A) Concentration determination in dependence of detected events. A serial dilution of EVs were measured with a concentration resulting in events within the dynamic range given by the manufacture (light red box). Left Y-axis, determined concentration, right Y-axis detected particles. Red line indicates threshold used in experiments. (B) Effect of EV particle input on staining rate output (positive events detection). Different amount of EVs (5 x 107; 1 x 108; 5 x 108; 1 x 109) were stained with PE-coupled anti-CD63 antibody at 2 ng/μL in a final volume of 100 μL freshly filtered PBS (0.1 μm ø). Detection of positive events decay when total number of particles was lower than 5 x 108. (C) Decrease of positive detection of additional tetraspanin markers. FITC-coupled anti-CD9 and anti-CD81 antibodies at 2 ng/μL were incubated with particles within the optimal (red bars) or sub-optimal (grey bars) range in a final volume of 100 μL freshly filtered PBS (0.1 μm ø). After staining, all samples were washed, ultracentrifuged and suspended in the same volume (50 µL) of freshly filtered PBS (0.1 μm ø) before fluorescence measurement by nFCM.
For this, we compared the detected number of events to the determined concentration, taking into account the dynamic range of the NanoAnalyzer according to the manufacturer's specifications (NanoFCM). The data show that with an event number at the upper threshold of the dynamic range, the concentration may be underestimated. With a range between 2000-10000 events/minute no large fluctuation in the determined concentration could be recognized, thus we assigned a dynamic range below 10000 events/minute.
To investigated whether the total number of EV particles has an impact on the positive-detectable CD63 events Therefore, we used an increasing amount of EVs from 5 x 107; 1 x 108; 5 x 108; and 1 x 109 particles as input for the staining procedure. EVs were incubated with PE-coupled anti-CD63 antibody at 2 ng/μL (in PBS) in a constant final volume of 100 μL. After 1 hour of incubation at 37 °C and a subsequent washing step followed by ultracentrifugation, we performed single-particle analysis by nFCM (Figure 2). In principle, the number of particles used as input should not affect the output, since it reflects the % of positive events detected in the total EV population. However, our results reveal that if particle input is reduced, the number of positive detectable events decreases. Beyond a certain particle concentration in the staining solution, saturation is reached at which no further increase in positive events can be detected (Figure 2A). This indicates that when staining EVs, an optimal quantitative range should be aimed at achieving maximal staining efficiency based on particle number. In our case, this range is around 5 x 108 particles for the antibody concentration used (here 2 ng/μL) to reach at least 80 % of the maximum possible staining for CD63 (Figure 2A). Furthermore, we can extrapolate this finding to other antibodies directed against other antigens. In addition to CD63, we observe a similar decrease in the detection of CD9 and CD81 when staining under optimal (> 109 particles) and suboptimal conditions (< 5 x 108 particles) from approximately 65 % to 40 % (0.65 relative change) and from 20 % to 10 % (0.61 relative change), respectively (Figure 2B).
It is worth mentioning that the detected positive rate (%) of the distinct antigens on the EVs may also vary depending on the antibody (clone) used. In addition, our findings only refer to EVs derived from ES-2 cells, whereas the phenotype of the different EVs can vary considerably between different cellular origins, even for canonical EV markers such as the tetraspanins [9, 11].
Particle concentration in the staining solution affects the % of positive detected events in an unexpected manner
To evaluate this question, we made an approach in which we examined whether the particle concentration in the staining reaction also influence the sensitivity and efficiency of detecting positive events. To this aim, we increased the reaction volume up to 1000 µL while maintaining the particle number (input) and antibody concentration constant, thereby, increasing the antibody : particle ratio (Table 1).
Table 1: Particle inputs and concentrations evaluated under different experimental conditions
Corresponding calculations for the experiment shown in Figure 3A are here provided. Particle concentration in the reaction tube seems to be critical for the optimal detection of positive events in an unexpected manner.
| Reaction volume (μL) | 50 | 100 | 200 | 500 | 1000 |
---|---|---|---|---|---|---|
Optimal Input (4 x 109 particles) | Particle concentration (No. particles/μL) | 8 x 107 | 4 x 107 | 2 x 107 | 8 x 106 | 4 x 106 |
Suboptimal Input (4 x 108 particles) | Particle concentration (No. particles/μL) | 8 x 106 | 4 x 106 | 2 x 106 | 8 x 105 | 4 x 105 |
This approach was replicated using two different particle input to determine the correlation between concentration and volume (Figure 3A).
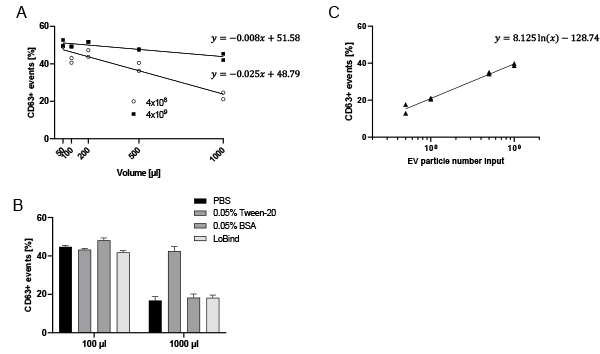
Figure 3: Effect of reaction volume and buffer conditions on positive fluorescence detection. (A) Effect of reaction volume on staining rate. A total of 4 x 109 (optimal, black squared dots) and 4 x 108 (suboptimal, white circle dots) EVs were stained with PE-coupled anti-CD63 antibody at 2 ng/μL in a final volume of 50, 100, 200, 500 or 1000 μL of freshly filtered PBS (0.1 μm ø). (B) Effect of different buffer composition on positive rate detection when working at different volumes under limiting conditions. A total of 4 x 108 EVs were stained with PE-coupled anti-CD63 antibody at 2 ng/µL in 100 µL of PBS, PBS + 0.05 % Tween-20, PBS + 0.05 % BSA or with PBS in LoBinding tubes. (C) Addition of 0.05 % Tween-20 during the staining procedure does not rescue the negative effect of low particle number. Different amount of input EVs (5 x 107; 1 x 108; 5 x 108; 1 x 109) were stained with PE-coupled anti-CD63 antibody at 2 ng/μL in a final volume of 100 μL freshly filtered PBS (0.1 μm ø) + 0.05 % Tween-20. Detection of positive events decay when total number of particles was lower than 5 x 108, as previously observed without Tween-20 (see Figure 1). After staining, all samples were washed, ultracentrifuged and suspended in the same volume (50 µL) of freshly filtered PBS (0.1 μm ø) before fluorescence measurement by nFCM.
Our results revealed that with increasing volume (decreasing concentration in the reaction), the detection rate declines in a linear manner. In particular, for the lowest number of particles stained (determined as suboptimal, c.a. 4 x 108 particle input, less than 4 x 106 particles/µL in the staining reaction), we were able to determine a decrease of almost 50 %. However, this effect becomes not that obvious when using 10-fold higher concentrated reactions (perceived as optimal, > 109 particles input, more than 4 x 106 particles/µL in the staining reaction).
Although unidentified stoichiometric effects cannot be dismissed, we hypothesize that our observations may respond to the “sticky” nature of EVs, which has been already described for some cellular models [8]. Our hypothesis is based on the possibility that EVs may stick to the tube during the staining procedure, therefore, determining a critical number of particles (threshold limit) to “coat” the tubes and to allow the rest of the EV population to be “accessible” to the antibody detection. That would explain why the decay on positive detection is only noticeable when working under suboptimal conditions (here determined as below 5 x 108 particles) in which particle concentration particularly decreases in the reaction volume (< 4 x 106 particles/µL, Table 1). Under these conditions, EVs would be more prone to stick to the tube and less accessible to the antibody, despite antibody : particle ratio notably increases.
To elucidate this question, we performed a third experiment in which we tested if different tubes/staining conditions affect the staining/detection efficiency. We compared two different reaction volumes based on only PBS, PBS + 0.05 % Tween-20, PBS + 0.05 % BSA and only PBS incubated in low binding protein tubes (Protein LoBind Tubes, Eppendorf, Hamburg, Germany). Antibody concentration (2 ng/µL), as well as particle input, was maintained (Figure 3B). We consciously worked under suboptimal input conditions (4 x 108 particles per tube) to make a potential “rescue” effect more pronounced, which would be likely not detectable using higher particle concentrations. Our results showed that if the reaction volume is low (100 µL), no differences according to the buffer composition or type of tube could be observed. However, under suboptimal staining conditions (1000 µL with low number of particles), only PBS + 0.05 % Tween-20 as staining solution resulted in a recovery of the positive detection output (Figure 3B). Our potential explanation is that a mild detergent concentration would avoid the EVs stick to the tube, making the particles more accessible for antibody detection and, therefore, increasing the output of positive events detected by nFCM. Nevertheless, if the number of particles does not reach the threshold (here determined as 5 x 108 particles), the underestimation of positive events is still observed despite the presence of detergent in the staining reaction buffer (Figure 3C).
We deem it essential to mention that all stained particles were subjected to a washing step followed by ultracentrifugation to collect stained particles. No significant differences in particle recovery were observed between conditions, therefore differences in output cannot be attributed to particle loss during the procedure and subsequent measurement. It should be noted that these results appear contradictory, as the stickiness of EVs could not only be unfavorable for antigen-antibody detection but also for EV recovery. In this regard, previous studies have shown that the EV isolation method, as well as the choice of plastic ware, may effect EV recovery [10]. Moreover, nFCM results presented here only refer to positive/negative detected events. Mean fluorescence intensity or any other sensitivity-related parameters have not been analyzed, since they were out of the scope of our work and would require further controls and experimental conditions.
Immunoblotting as a proof-of-concept: particle number as a critical parameter also for intravesicular marker detection
To analyze intravesicular EV markers (i. e., Alix or TSG101), we also performed classical immunoblotting assays as recommended by MISEV guidelines [12]. Despite immunoblotting is a widely used technique, sensitivity of antibodies and determination of the dynamic range of signal detection are common challenges that can be also influenced by the method of detection [13]. Here, we provided a representative immunoblot detecting intravesicular markers (Alix, FLOT-1, TSG101) based on the total particle number as input (Figure 4A).
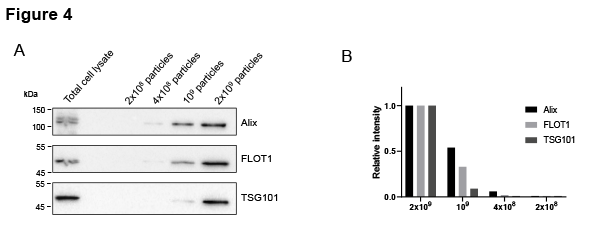
Figure 4: Western blot analyses for intravesicular markers. (A) Detection of well-known intravesicular markers as Alix (98 kDa), FLOT-1 (47 kDa) and TSG101 (44 kDa). Different amounts of particles were lysed in RIPA 10X buffer and resolved on SDS-PAGE (10 % gels). Loading was based on particle number instead of protein content. Total cell lysate (15 μg) was loaded as positive signal control. (B) Western blot semi-quantitative results. Relative intensity values obtained after densitometry of the bands (graph bars) by Image Lab software.
Our results showed that at least 109 particles must be loaded, although with differential sensitivity depending on the antibodies/markers tested. As an example, only anti-Alix (1 : 500 dilution) showed two values within the dynamic range of detection (black bars, Figure 4B) indicating that loading of more than 109 particles should be considered. In direct comparison, the estimated suboptimal particle number for nFCM (4 x 108 particles) is not a choice for immunoblotting, underlying the greater sensitivity of single-vesicle fluorescence analyses. To our knowledge, immunoblots based on particle number has not yet been reported by others and therefore we highly encourage to do this. Especially considering that EV samples are very heterogeneous and protein contamination depend strongly on the purification method [12]. Furthermore, canonical normalization markers probably cannot be determined. Despite these observations, optimization of particle number depends on the marker of choice and should be implemented for every type of sample. However, we strongly believe that particle number should be used as a normalizer when comparing different conditions. Although still naive, we hope our results pave the way providing some data to be used as a reference in future research.
Concluding Remarks
Our starting experimental setting for the staining protocol (antibody concentration, 2 ng/μL and reaction volume, 100 μL) was based on previous references for FC evaluation of EVs. We assumed that under these experimental conditions our antibody is present in a non-limiting amount (200 ng) and, therefore, should be enough to detect any positive event on the sample reaction, especially if the number of positive events (in absolute terms) is reduced and therefore the antibody : particle ratio increases. However, no proper particle number titration had been described so far and parallel experiments in our group pointed out a tendency of decreased positive detection especially in low EV yield samples (i. e., derived from primary cells) (data not shown). In addition, the “stickiness” of EVs from different origin and isolation method has been recently pointed out [14] and this natural occurring peculiarity may interfere with the increasingly sensitive techniques. In particular, the concept of an EV-enveloping corona should be noted here, which may explain the various molecular interactions on the surface of EVs with their environment [15].
In summary, our results underline again the importance of standardization and determination of the optimal conditions before conclusions are reached. These questions appear to be now indispensable, especially when using more sophisticated, sensitive techniques for analyzing EVs.
Material and methods
Cell culture
ES-2 cells were maintained at 37 °C in 5 % CO2 in Dulbecco’s Modified Eagle Medium (Gibco™, Thermo Fisher Scientific) supplemented with 10 % fetal bovine serum (Gibco™, Thermo Fisher Scientific). For EV production, 1 x 107 cells were seeded per T175 flask (Greiner Bio-One). After 24 h medium was removed and cells were washed with 1 x PBS, followed by adding 25 mL CD293 medium (Gibco™, Thermo Fisher Scientific) supplemented with 1 % penicillin-streptomycin and 1 x GlutaMAX™ (Gibco™, Thermo Fisher Scientific), cells were cultured for 48 h.
Isolation of extracellular vesicles
EVs were isolated from the cell culture medium by differential centrifugation at 400 xg for 5 minutes; 2,000 xg for 10 minutes and 10,000 xg for 30 minutes at 4 °C. Supernatants were ultra-filtrated using centrifugal filters (Amicon Ultra-15, 10 kDa) to a final volume of approximately 500 µL. Subsequently, the concentrated EV samples were loaded onto a size-exclusion column (Izon Science), which were pre-equilibrated with 1 x PBS. Fractions of 0.5 mL were collected and the fractions (7–9) which correspond to the known retention volume of EVs, were pooled and concentrated to 50 µl by ultracentrifugation at 110,000 xg for 2 hours using an OptimaTM MAX-XP ultracentrifuge (TLA-45 fixed-angle rotor, Beckman Coulter, Inc.).
Electron microscopy
EVs were prepared for electron microscopy as described previously (Théry et al., 2006) with little modifications. The purified EVs were fixed with an equal amount of 4 % PFA. A small volume (5–7 µl) of this suspension was transferred to a formvar/carbon-coated meshed copper grid (Ted Pella Inc., Redding, CA) and air-dried for 20 min. The grids were washed with sterile-filtered PBS and fixed for 5 min with 1 % glutaraldehyde. After 8 washing steps (2 min each) with distilled water, the sample was incubated with 1 % uranyl acetate for 5 min and then transferred to 2 % methylcellulose supplemented with 4 % uranyl acetate (ratio 9 : 1) and incubated for 10 min on ice. Excess fluid was removed and the grid was air-dried for up to 10 min. Images were taken with a Zeiss EM 900 at 80 kV equipped with a 2k slow-scan CCD camera (TRS, Moorenweis, Germany).
Nano flow cytometry measurements
For nano flow cytometry of isolated EVs, a Nanoanalyzer (NanoFCM Co. Ltd., Nottingham, UK) equipped with a 488 nm laser and two single photon-counting avalanche photodiodes (ADP) was calibrated using 200 nm polystyrene beads (NanoFCM Co. Ltd.), with a defined concentration of 2.08 x 108 particles/mL. In addition, monodisperse silica beads (NanoFCM Co. Ltd.) of four different sizes (68 nm, 91 nm, 113 nm, 155 nm) were used as a size reference standard. Freshly filtered (0.1 µm) 1 × phosphate-buffered saline (PBS) was analyzed as background signal and subtracted from the other measurements. Each distribution histogram or dot-plot was derived either from data collected for 1 min (particle concentration) or after detecting approximately 4000 events (fluorescent measurements), with a sample pressure of 1.0 kPa. The EV samples were diluted with filtered (0.1 µm) 1 × PBS, resulting in a particle count in the optimum range of 2,500–12,000 events. Particle concentration, size distribution and % of fluorescence positive events were calculated using the NanoFCM software (NF Profession V1.08, NanoFCM Co. Ltd.). For immunofluorescent staining, the following antibodies were used: FITC-conjugated mouse antihuman CD9 antibody (clone HI9a, Cat. 312104, BioLegend, Koblenz, Germany), FITC-conjugated mouse antihuman CD81 antibody (clone 5A6, Cat. 349504, BioLegend), PE-conjugated mouse antihuman CD63 antibody (clone H5C6, Cat. 353003, BioLegend).
Immunoblotting
EV samples were homogenized in RIPA (10X) solution by vortexing and incubation on ice for 10 min followed by the addition of Laemmli buffer (5X - 255 mM Tris-HCl, 50 % glycerin, 5 % (w/v) SDS, 250 mM DTT, 0.05 % (w/v) bromophenol blue) and samples were subsequently incubated for 5 min at 95 °C. Samples were run on a 10 % SDS protein gel and transferred to Hybond ECL nitrocellulose membranes according to standard procedures. Membranes were blocked for 2 h with 3 % (w/v) BSA in TBS buffer and probed O/N at 4 °C at 1 : 500 dilution in 3 % (w/v) BSA in TBS buffer containing 0.2 % Tween-20 (TBS-T) using the following antibodies: mouse anti-Alix (Cat. sc-53540, Santa Cruz Biotechnology, Heidelberg, Germany), anti-FLOT-1 (Cat. 610821, BD Biosciences, San Jose, USA) and anti-TSG101 (Cat. sc-7964, Santa Cruz Biotechnology). Blots were washed and incubated with anti-mouse IgG-HRP-conjugated secondary antibody (Cat. 7076S, Cell Signalling, Frankfurt, Germany) for 1h at 1 : 10000 dilution in TBS-T. The immunoreactive bands were incubated with Immobilon Forte Western HRP substrate Millipore (Merck, Darmstadt, Germany) and visualized using a ChemiDoc MP system (Bio-Rad, Hercules, CA, USA). Blots were exposed at different times. Optical densities of the immunoreactive bands were measured using Image Lab analysis software version 5 (Bio-Rad). Over-exposed bands were excluded from the relative quantification.
Funding
This study was supported by the Deutsche Forschungsgemeinschaft (DFG, German Research Foundation) - 416910386 - GRK 2573/1.
Acknowledgement
We thank Dr. Frederick Helmprobst from the Mouse Pathology and Electron Microscopy Core Facility at the University Marburg for support.