Elucidating the role of extracellular vesicles in the Barley-Fusarium interaction
Fusarium graminearum (Fg) is a necrotrophic fungal pathogen that causes devastating diseases on its crop hosts barley and wheat. Recently, small RNAs (sRNAs) were identified as mobile communication signals between eukaryotes and their pathogens, symbionts or parasites. It has been shown that pathogens secrete sRNAs as effectors to suppress plant immunity and plants use endogenous sRNAs to resist infection, a phenomenon termed cross-kingdom RNAi; ckRNAi. However, little is known about the transport of fungus- or plant produced sRNAs to silence genes that contribute to immunity.
Keywords: Fusarium graminearum, Barley, plant EV, fungal EV, ckRNAi
Introduction
Fusarium graminearum (Fg) is one of the most important cereal killers worldwide that causes devastating diseases of crops with great economic and agronomic impact on global grain industry [1,2]. In addition to yield losses, food quality is affected by grain contamination with mycotoxins, which are produced by the fungi during plant infection, representing a serious threat to human and animal health [3,4]. Plant protection and toxin reduction strategies currently rely on the application of systemic fungicides [5]. However, many plant pathogenic fungi have become less sensitive or even resistant to chemical treatments. Thus, alternative control strategies have been developed that may lead to long-term reduction of pesticide use in modern agriculture.
RNA interference (RNAi; also known as RNA silencing) is a conserved mechanism for the regulation of gene expression in eukaryotes. RNAi is mediated by small RNAs (sRNAs) directing gene silencing at the transcriptional or post-transcriptional level. Transcriptional gene silencing results from epigenetic modifications, specifically DNA methylation and histone modifications regulating heterochromatin formation [6,7]. Post-transcriptional gene silencing starts with the cleavage of a precursor double-stranded (ds)RNA into short 21–24 nucleotide (nt) microRNAs (miRNAs) or small interfering RNAs (siRNAs) by type-III RNA endonuclease Dicer-like proteins (DCLs). Subsequent binding of siRNAs to an Argonaute protein (AGO) lead to the formation of the RNA-induced silencing complex (RISC) that mediates targeting of complementary mRNAs for degradation or translational inhibition [8,9]. Plants have evolved RNAi pathways/machineries that not only direct the epigenetic regulation of their own genome, but also provide protection from invasion by foreign nucleic acids, such as viruses [10]. Our laboratory and other groups previously demonstrated that this natural phenomenon can be used to control agronomically relevant plant diseases, based on the demonstration that in planta expression of dsRNA (termed host-induced gene silencing; HIGS; [11]) as well as exogenous spray application of dsRNA (termed spray-induced gene silencing; SIGS; [12]) can signal post-transcriptional gene silencing of target genes in various plant pathogens and pests (for review see: [13–19]).
Despite these findings, recent studies revealed RNAi between kingdoms as a new level of inter-species communication designated as cross-kingdom RNAi (ckRNAi). This phenomenon was first described by Arne Weiberg and Hailing Jin in 2013, demonstrating that the fungal pathogen Botrytis cinerea produces sRNAs that mimic plant sRNAs and bind to Arabidopsis AGO1 to antagonistically silence important plant immunity genes [20]. Since then, several studies have demonstrated bidirectional sRNA trafficking between plant hosts and their interacting pathogens [21,22]. However, the mechanisms underlying the transfer and uptake of ckRNAi-related sRNAs as well as transgene-derived artificial sRNAs (HIGS) remained unknown for a long time. It has been proposed that extracellular vesicles (EVs) playing a key role in the bidirectional transfer of sRNAs that mediate ckRNAi [13,21,23,24]. EVs are surrounded by a lipid bilayer and can be differentiated by size and their process, thus, classified in three major groups: The largest EVs are 5000-1000 nm in diameter known as apoptotic bodies, which are generated during cell lysis. Microvesicles are 1000-100 nm in size and produced by cells during exocytosis. The third class are exosomes which measure 150-30 nm and are released by fusion of multivesicular bodies with the cell membrane [25]. Plant EV research is an emerging field, so far only two reports described the isolation of plant EVs and the characterization of their protein/RNA content [26,27]. Therefore, lots of questions and methodological challenges need to be addressed to reach the quality standards in mammalian EV research. For example, identification of suitable plant EV markers as well as developing strategies that increase the purity of EV isolates, necessary “to distinguish bona fide EV cargo from merely co-purifying contaminants” (for more details see: [28]). However, in plant EVs were firstly discovered in 1967 using electron microscopy [29]. Sixty years later Rutter and Innes successfully isolated the first EVs from apoplastic washes of the model plant Arabidopsis thaliana (Arabidopsis) [26]. The authors demonstrate that Arabidopsis EVs were enriched in proteins responsible for abiotic and biotic stress response which indicates an important role of EVs in plant immune responses. Moreover, in this study the first plant EV protein marker, the syntaxin PEN1 (PENETRATION 1) was described.
Recently, we showed that EVs purified from Arabidopsis leaf extracts and apoplastic fluids contain transgene/HIGS-derived sRNAs [27]. To our knowledge, this was the first report demonstrating sRNAs as plant EV cargo. In addition, we found that mutants of the ESCRT-III complex (ENDOSOMAL SORTING COMPLEX REQUIRED FOR TRANSPORT III) were impaired in HIGS further indicating that endosomal vesicle trafficking supports transfer of transgene-derived siRNAs between donor host cells and recipient fungal cells. Regarding the possibility of ckRNAi in Fg, we analyzed sRNA profiles of Fg axenic cultures and identified Fg sRNA target genes in barley and the monocot model plant Brachypodium distachyon. Subsequent expression analysis revealed that Fg-derived sRNAs caused significant downregulation of two host defense-related genes (unpublished data). Moreover, we recently demonstrated that targeting fungal DCL genes (FgDCL1 and FgDCL2) via SIGS could protect barley leaves from Fg infection [30]. Together, our recent results point to the direction that ckRNAi exists in the Fg – barley interaction. Supporting this notion and to further investigate whether Fg utilizes EVs for the transfer of sRNAs that manipulate and dampen immunity of its host barley, we isolated EVs from Fg liquid culture and performed Fg EV infiltration of barley leaves. In addition, we conducted an in vitro growth experiment, in which we isolated EVs from barley leaves to test their impact on fungal growth regarding the possibility of bidirectional ckRNAi in Fg-barley interaction.
Material and Methods
Plant cultivation and barley EV isolation
Barley (Hordeum vulgare cultivar Golden Promise) plants were grown for 14 days with 16 h light per day at a temperature rhythm of 18 °C/14 °C (day/night) and a humidity of 65 %. 80 leaves were taken for EV isolation. EVs were isolated as previously described [27]. EVs were resuspended in 190 µl phosphate buffered saline (PBS; 140 mM NaCl, 2,5 mM KCl, 10 mM Na2HPO4, 2 mM KH2PO4, pH 7,4) for further analysis.
Co-incubation assays of barley EVs with Fg
400 µl Fg IFA65 macroconidia with a concentration of 30.000 macroconidia per millilitre were sprayed on 0.5 x PDA (potato dextrose agar) plates. One hour after spraying, 40 µl of barley EV suspension was dropped into the middle of each agar plate. Plates were incubated for six days at room temperature (RT) under constant illumination from one near-UV tube (Philips TLD 36 W/08) and one white-light tube (Phillips TLD 36 W/830HF).
EV isolation from Fg liquid cultures
For EV isolation, Fg was grown for four weeks in synthetic nutrient poor broth (SNP) containing 1.5 % carboxymethylcellulose (CMC) at 28 °C with 180 rpm shaking. Upon cultivation, fungal suspension was filtered through a miracloth mesh and centrifuged at 10,000 g for 20 minutes. The supernatant was filtered subsequently through a 0.45 µm and a 0.22 µm polyvinylidenfluorid (PVDF) membrane. EVs were concentrated ten times by tangential flow filtration (TFF) and centrifuged for 22 hours at 150,000 g at 4 °C. Afterwards, the pellet was resuspended in PBS buffer and loaded on the top of a discontinuous sucrose gradient with fractions of 8 %, 16 %, 35 %, 45 % and 60 % sucrose. The 30 %, 45 % and 60 % sucrose fractions were separated after centrifugation and resuspended in PBS buffer and centrifuged again by 4 °C at 150,000 g. Finally, the pellet was resuspended in a small amount of PBS buffer for nanoparticle tracking analysis (NTA), transmission electron microscopy (TEM) and plant infiltration assays.
Measuring vesicle size and concentration by nanoparticle trafficking analysis (NTA)
For NTA, purified barley and Fg EVs were diluted (1:50) with PBS buffer. Subsequently, 500 µl of vesicle suspension was loaded into Nanosight NS300 (Malvern Panalytical) and five measurements were performed at 25 °C. Size and concentration predictions as well as statistical analysis were performed using NTA 3.2 Dev Build 3.2.16 software.
Negative staining and transmission electron microscopy (TEM)
For TEM, five µl of purified EVs were loaded on copper formvar-coated 300-mesh electron microscopy grids, which were glow discharged prior to sample application for 40 sec. Redundant liquid was swabbed by using Whatman filter paper. Grids were incubated three times with 50 µl of 2 % uranyl acetate and washed with distilled water. Grids were air dried. Preparations were inspected at 120 kV under zero-loss conditions (ZEISS EM912a/b) and images were recorded at slight under focus using a cooled 2k × 2k slow-scan ccd camera (SharpEye / TRS) and the iTEM software package (Olympus-SIS).
Barley infiltration assays
For infiltration, the resuspended Fg EV pellet was adjusted to a final volume of 500 µl with PBS buffer. The downside of leaves from nine-day old barley (Hordeum vulgare cv. Golden Promise) and five-weeks old Nicotiana benthamiana plants was pressure-infiltrated with 500 µl of the Fg EV suspension/solution using a 1-ml syringe. After five days post infiltration leaves were photographed and harvested for further analysis.
Results and Discussion
Barley EVs affect Fg growth and pigmentation
In this study, barley EVs were purified from the second and third leaf of two-weeks old plants and were subjected to quality control using NTA and TEM analysis. NTA revealed 2.0 x 109 particles per millilitre that were isolated from 80 barley leaves with a mean size of 156 nm and a mode size of 165 nm (Tab. 1; Fig. 1A).
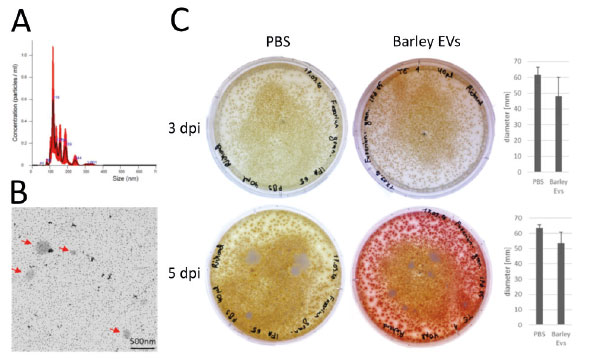
A clear peak was visible for particles with 119 nm in diameter, which agreed with the size range reported for EVs from mammalian cells (30–150 nm) [31] as well as plants (50–300 nm) [23]. Almost no particles were measured above 350 nm and below 80 nm (Fig. 1A). In TEM analysis, particles negatively stained with uranyl acetate showed circular EV-like structures (Fig. 1B), as previously observed [27].
To test whether barley EVs have an impact on fungal growth, we drop-inoculated isolated EVs onto Fg culture plates. As mock control, PBS buffer was dropped on Fg plates. At six days post inoculation (dpi), different colony density at the drop-inoculated colony centre was found compared to the external area of the colony (Fig. 1C). We further observed that the colony density and pigmentation changes gradually, with the marginal colonies mainly produced white mycelium (Fig. 1C). The area of denser colonies was the same for both treatments (Fig. 1C), indicating that this effect was caused by the PBS buffer and not by the purified plant EVs. Besides this, PBS treated Fg colonies did not show any change in pigmentation (Fig. 1C). Barley EV-treated colonies underwent a change in pigmentation dependent from the distance to the EV inoculation spot. They turned from yellow (close to the inoculation) to purple the further away from the centre of the plate (5dpi; Fig. 1C). The change in pigmentation was independent from the amount of drop inoculated EVs. We suggested that barley EV treatment caused the change in pigmentation of Fg cultures. One possibility could be that the premature purple pigmentation was a stress response, which was induced by the EV treatment. Accumulation of blue-violet pigments is found in peridermal cells of Fg perithecia (fruiting bodies) [32], thus, we assumed an early switch into the reproductive/sexual lifecycle upon EV treatment. Further experiments would be necessary to test this hypothesis. Moreover, whether the changes in Fg pigmentation were caused by specific effects related to an unknown barley EV cargo or represented an unspecific effect due to the presence of plant EVs per se or co-purified contaminants needs to be assessed. Consistent with our results on Fg growth inhibition, a previous study demonstrated that EVs from sunflower seedlings affected the growth of Sclerotinia sclerotiorum spores, suggesting EV-mediated secretion of antifungal compounds [33]. Supporting this, Arabidopsis EVs were also shown to contain defence-related cargo molecules, such as antimicrobial glucosinolates, proteins for oxidative stress response and reactive oxygen species (ROS) signalling [26,27]. However, further studies are needed to elucidate the role of plant EVs in plant-fungal interaction and the underlying molecular mechanism of EV loading and sorting in the donor plant cells as well as EV sensing, uptake into the recipient fungal cells.
EVs are produced by Fg
Whereas the isolation and characterization of mammalian EVs is well established, isolation of EVs from plants and plant-infecting pathogens is less advanced. To test whether Fg ckRNAi involves EVs as communication vehicles we conducted an experiment of infiltrating Fg EVs into barley leaves. We developed and optimized a protocol for EV isolation from Fg liquid cultures (see Material and Methods). To avoid EV contaminants from nutrient broth ingredients, we choose a synthetic nutrient-poor broth containing carboxymethylcellulose (CMC) as a solid compartment to allow fungal growth which only takes place attached on solid phases. The most particles counted by NTA were in the fraction (F) of 45 % sucrose with 1.97 x 1010 particles per millilitre (ml) compared to 1.18 x 1010 particles/ml for F 30 % and 1 x 1010 particles/ml for F 60 % (Tab. 1).
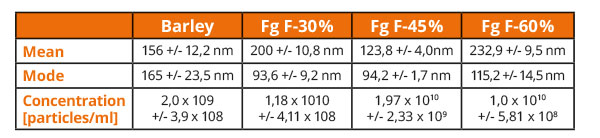
The particle size distribution for F 45 % was more homogenous compared to the F 30 % and F 60 % fractions (Fig. 2A).
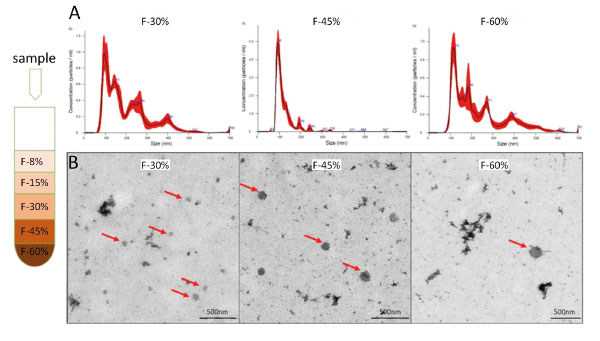
Moreover, F 45 % showed a distinct peak for particles with a size of 95 nm and only low abundance of particles of other sizes (Fig. 2A; Tab.1). The mean size of F 45 % was 123 nm and the mode size 94 nm, which was comparable with the size range reported for EVs from human fungal pathogens (~100 nm) and plant pathogenic fungi (150-155 nm) [34]. Notably, the F 30 % also contained a high number of particles with 95 nm in size, suggesting an overlap of the same EV population, however, particles with > 200 nm sizes were co-isolated in this fraction (calculated mean particle size of 200 nm and a mode size of 93 nm) (Tab. 1). F 60 % showed particles with a mean size of 232 nm and a mode size of 115 nm (Tab. 1). Consistent with our findings, previous studies also described a size range of EVs isolated from filamentous fungi, e. g. Alternaria infectoria (20-40 nm) [35] and Trichophyton rubrum (20-380 nm) [36]. Variation in EV size distribution was also described for the human fungal pathogens, Candida albicans and Candida neoformans [37], suggesting a heterogenous nature of fungal EV populations. Whether this correlates with a functional diversification of EVs in fungi per se remains to be elucidated. To confirm NTA data and to study fungal EV morphology, samples from F 30 %, F 45 % and F 60 % were fixed on a cupper mesh and negatively stained with uranyl acetate to perform TEM. Interestingly, TEM analysis revealed cup shaped EV structures in all three fractions. Consistent with NTA results, EVs from F 45 % were homogenous in size and appearance, while EVs from F 30 % and F 60 % varied in size and electron density indicating different EV composition and subpopulations (Fig. 2B).
Infiltration of Fg EVs caused phytotoxic effects in barley leaves
Our recent data pointed to the direction that Fg probably utilizes sRNAs as virulence factors ( [30]; unpublished). To further support this finding and to study whether this ckRNAi was mediated by EVs, we conducted an experiment of infiltrating Fg EVs into barley leaves. Barley (host) as well as Nicotiana benthamiana (non-host) leaves were pressure-infiltrated with 500 µl of each EV fraction, F 30 %, F 45 % and F 60 %. To exclude unspecific effects caused by the infiltration procedure or the EV resuspension buffer, water and PBS were infiltrated as controls. In addition, Fg macroconidia were infiltrated to test whether barley leaves respond to foreign, pathogenic material itself. Finally, fungal culture supernatant after concentration by tangential flow filtration was infiltrated to assess whether the crude EV extract cause any damage on barley leaf tissue. The infiltration of water and fungal spores caused no effects on plants, whereas some barley leaves that were infiltrated with PBS showed small yellow spots restricted to the infiltration site. However, it was unclear, if this was due to PBS or resulted from the harsh infiltration procedure. Of note, barley leaves infiltrated with Fg EVs exhibited phytotoxic response observed as leaf bleaching and discoloration that appeared around the infiltration area (Fig. 3A).
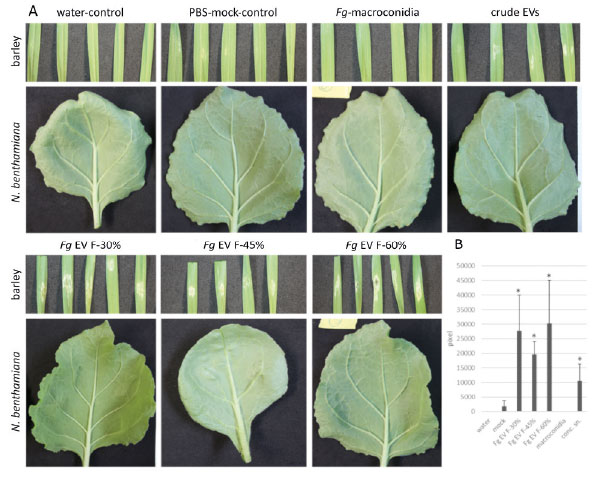
Importantly, we observed that these phytotoxic effects were independent of the Fg EV fraction that was used for infiltration (Fig. 3B). Remarkably, the infiltration of concentrated culture supernatant led to the formation of bleached spots as well, however, they were much smaller compared to those observed for the Fg EV fractions (Fig. 3B). This result may indicate a dose-dependent effect in barley, because the culture supernatant contained less EVs (diluted in a higher volume) compared to the concentrated Fg EV fractions. However, these results were consistent with another study demonstrating phytotoxic effects of Fusarium oxysporum f. sp. vasinfectum EVs on cotton plants [34]. Interestingly and in contrast to our results the authors found that infiltration of resuspended spores and hyphae led to the formation of discoloured spots as well, even if they were smaller compared to the EV infiltration. Moreover, the authors demonstrated that phytotoxic effects were not host specific as EV infiltration of the non-host plant N. benthamiana caused similar phenotypes. We observed contrasting results for N. benthamiana, as neither the infiltration of water, spores, PBS, EV fractions nor concentrated culture supernatant caused any effect on the tobacco leaves (Fig. 3A), indicating species specificity of EV cargo in the genus Fusarium. Therefore, further analysis of Fg EV cargo (protein and RNA) should clarify the role of fungal EVs in the infection process and to unravel the molecular mechanisms of EVs underlying the ckRNAi phenomenon.
Acknowledgement
We thank C. Birkenstock for excellent plant cultivation. C. Preußer and M. Mosbach for helping with the NTA measurements. M. Hardt and A. Möbus for the support during TEM sample preparation and microscopy. This work was supported by the Deutsche Forschungsgemeinschaft, Research Training Group (RTG) 2355 (project number 325443116) to TS and AK.