Extracellular vesicles isolation and standardisation
Isolation and characterization of extracellular vesicles
DOI: https://doi.org/10.47184/tev.2019.01.02Since EVs are secreted by most, if not all, eukaryotic and prokaryotic cells, they have been detected in body fluids as diverse as blood, urine and saliva as well as in cell culture media. This manuscript gives an overview of EV isolation and characterization strategies and highlight their advantages and disadvantages.
Keywords: extracellular vesicle isolation, purification, characterization, exosome
EV isolation: finding the needle in the haystack
The fundamental goal of a successful isolation method entails both the efficient enrichment of EVs from a given biofluid and the depletion of non-EV material, yielding preparations of high purity without significant loss of EVs (Figure 1).
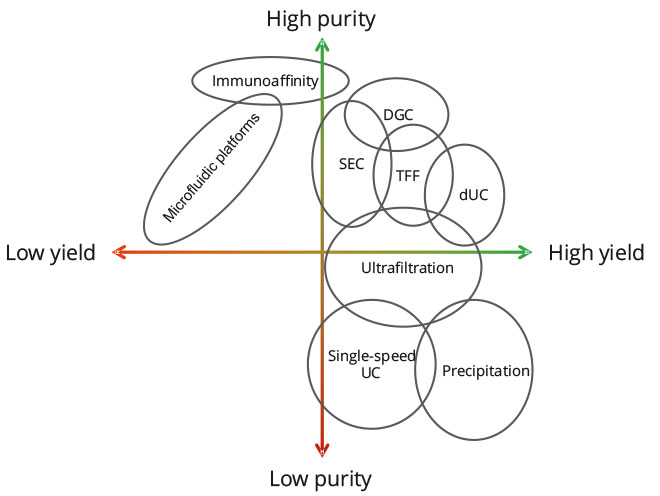
To this end, some approaches utilize principles that tackle general EV characteristics including size, density or charge, while others hone in on more specific attributes such as marker protein expression. Although enriching EVs itself is not trivial, separating them from contaminants such as protein complexes, aggregates and lipoproteins, which oftentimes share biophysical properties with EVs, is still a major challenge in the field. Each biofluid presents its own difficulties, ranging from high protein content (e.g. milk) to low EV concentration (e.g. urine) or lipoproteins that outnumber EVs by an order of magnitude (e.g. plasma).
Sedimentation-based methods
The most conventional method of EV isolation, which is still considered to be the gold standard by many, is differential ultracentrifugation (dUC) [1]. During multiple rounds of centrifugation with increasing speed, biofluids are initially depleted of large components before pelleting EVs at speeds of at least 100,000 g. Given sufficient run time, dUC is an effective enrichment method, but the resulting pellet will invariably contain non-EV contaminants that co-sediment alongside EVs and centrifugal force can lead to EV aggregation and damage.
Since EV preparations derived from sedimentation oftentimes do not display adequate purity for downstream assays, dUC can be combined with density gradient centrifugation (DGC). In this approach, the EV-containing ultracentrifugation pellet is resuspended and further fractionated by floatation into a density gradient. During centrifugation of the gradient, which is commonly prepared using sucrose or iodixanol, EVs will migrate until reaching a position where their density matches that of the density matrix. Individual density fractions are collected, concentrated by ultracentrifugation or filtration and utilized for downstream experiments. While there are various DGC protocols, including continuous or stepped gradients as well as top-down and bottom-up floatation, the underlying principle of density-based fractionation is identical. Even though the combination of dUC with DGC provides orthogonal separation based on sedimentation and density, it does not achieve absolute EV purity, since densities of common contaminants such as high-density lipoproteins overlap with those of EVs [2].
Method | Principle | Advantages | Disadvantages |
dUC | Sequential separation of EVs and other biofluid components based on their sedimentation rate and density | High EV yield, established protocols, medium to large sample volumes, scalable | Time-intensive, high equipment cost, low portability, low purity, limited parallel sample processing, potential EV damage |
DGC | Improved purity compared to dUC, lends itself to combination with other method | Labor- and time-intensive, low yield, low throughput | |
Precipitation | Solubility of EVs is altered by water-excluding polymers or manipulation of EV surface charge | Quick and simple, large sample volumes, high yield, small to large sample volumes, low cost, scalable, parallel sample processing | Very unspecific, co-precipitation of non-EV contaminants, precipitation reagent needs to be removed (for some applications) |
Affinity | Interaction between specific EV surface molecules and capture antibodies | High purity, enables subtyping of EV populations, parallel sample processing, commercial kits and self-made protocols | Biased for specific populations, harsh or no elution, high reagent cost, limited scalability, small sample volumes |
(Ultra)filtration | EV isolation is exclusively based on the size differences between EVs and other particulate constituents | Quick and simple, portable, low to moderate costs, small to large sample volumes, parallel sample processing, | EV loss on membrane, potential clogging and EV trapping, shear stress |
lends itself to combination with other method | |||
SEC | Moderate to high purity, low physical forces preserve EV integrity, availability of commercial kits and self-made devices | Low to moderate equipment cost, small to moderate sample volumes, fractions might be sample-dependent | |
Microfluidics | Microscale isolation based on a variety of EV properties including immunoaffinity, size, and density | Isolation from minimal volumes, modifiable, portable, fast, easy automation and integration | Lack of standardization and validation, low to moderate throughput, expensive |
DGC: density gradient centrifugation; dUC: differential ultracentrifugation; SEC: size-exclusion chromatography |
Table 1: Comparative overview of commonly used EV isolation methods.
Filtration-based methods
Filtration-based isolation primarily depends on size and molecular weight differences between EVs, proteins and other components present in biofluids. Ultrafiltration is a size-based technique that utilizes membrane filters with a defined molecular weight or size exclusion limit to separate EVs from biofluids and potential contaminants. Transmembrane pressures that force the suspension through the membrane filters can be generated by pumps or centrifugation. Sequential filtration is often performed with filters that decrease in their pore size to first eliminate larger structures and then smaller compounds from the biofluid before EVs will be captured. Tangential flow filtration (TFF) has emerged to retain and thus concentrate EVs by concurrently decreasing the sample volume. In contrast to traditional ultrafiltration, TFF enables tangential fluid flow across the surface of a hollow fiber membrane, which prevents clogging of filters. Another common size-based separation technique is size-exclusion chromatography (SEC), also known as gel filtration. In SEC, EV samples are loaded on a column prefilled with a porous stationary phase (e.g. sepharose). Small particles can enter the pores of the stationary phase, leading to increased retention time and delayed elution compared to larger particles that are excluded from entering the pores. Using specialized columns, this method allows the separation of EV size-defined subtypes as individual populations.
Affinity-based methods
Continued advances in characterizing the molecular composition of EVs also opened new avenues for their isolation. Once specific proteins, lipids or carbohydrates on the EV surface are identified, they can be targeted by appropriate capturing receptors immobilized on beads or membranes. During this affinity-based isolation, EVs are purified by selective receptor-ligand interaction, potentially resulting in high specificity and purity.
Some of the first affinity-based isolation methods focused on common EV-associated tetraspanins such as CD63, CD81 or CD9 [3]. Since these transmembrane proteins display readily accessible domains on the EV surface, they lend themselves to capture by specific antibodies. Common immunoaffinity-based isolation protocols utilize a mix of antibodies to capture a wide range of EVs that display these markers. An alternative approach, which is of significant interest for diagnostic applications, might target surface proteins or glycan structures that are only expressed in specific tissues. Alternatively, a robust, disease-specific marker that is exclusively presented on EVs from the affected tissue, potentially allows specific capture of relevant EV subpopulations [4].
The interaction with surface targets is not limited to antibodies: successful isolation has been reported based on using lectins, phosphatidylserine binding proteins and synthetic peptides to capture EVs. Less specific approaches include isolation based on affinity to charged membranes or capturing EVs using heparins, which bind to several EV surface proteins.
As any other method, affinity-based isolation introduces bias since it very likely only captures a subset of all EVs in a given sample. While their selectivity potentially results in favorable purity and signal-to-noise ratio, the dependency on high-affinity antibodies, substantial reagent costs and inability to elute captured EVs limit many of the affinity-based isolation methods available today.
Precipitation-based methods
Originally, the precipitation technique was established to separate and concentrate viruses. Since viruses and EVs share biophysical properties, precipitation techniques have been adapted for the enrichment of EVs. In general, EV precipitation relies on manipulating their solubility and dispersibility in aqueous suspensions. The most common precipitation technique is the polymer-based precipitation. Hydrophilic polymers such as polyethylene glycol (PEG) tie up water molecules, which leads to the concentration of EVs until solubility is exceeded and precipitation occurs. Since EVs are negatively charged, polymer solutions can be supplemented with positively charged protamine sulphate in charge-based precipitation approaches or used in combination with dextran in a two-phase-system. ‘Salting-out’ allows immediate EV precipitation due to EV charge neutralization with acetate ions. After the precipitation process is completed, EVs can be pelleted by low‐speed centrifugation. In addition, indirect EV precipitation approaches have been developed that first precipitate soluble proteins present in biofluids with organic solvents. After centrifugation, EVs can be isolated from the pre-cleared supernatant by the method of choice.
Fluidics-based methods
Microfluidic platforms for EV isolation from particularly small sample volumes attracted the attention of researchers and clinicians in the last years. In general, these miniaturized tools follow the same principles as large-scale isolation methods. For example, EV-targeting antibodies can be immobilized on solid surfaces of microfluidic devices. Oligonucleotide and peptide aptamers offer an alternative to antibodies and both allow affinity capturing and produce a reporter signal upon EV binding. Membrane-based filtration approaches and viscoelastic flow sorting have been also implemented in microfluidic isolation procedures. Alternative microscale systems are nanotraps that consist of nanowires in 3D-networks that bind to and capture EVs from various biofluids. Other devices have implemented innovative sorting mechanisms such as acoustic trapping (e.g. by ultrasound) or electrophoretic and electromagnetic manipulation for EV isolation [5].
Asymmetric-flow field-flow fractionation (AF4) is a hydrodynamic method at microscale level that separates EVs based on their differences in size and diffusivity [6]. Samples are applied to a miniature fluidic channel, in which two orthogonal flows control EV separation. A laminar parabolic flow (i.e. fastest flow in the center, slowest flow at the walls) runs along the channel axis and transports the sample towards the detector. The second flow is perpendicular to the laminar flow and vertically distributes particles against the chamber wall. Smaller particles diffuse further from the flow chamber wall and are eluted earlier than larger ones. AF4 can be integrated with various types of detectors depending on the information required.
EV characterization:
defining the needle
Analytical characterization of EV preparations is a multi-step process that serves several purposes. First, it is important to detect EVs in order to verify that a successful isolation has been performed. Next, quantifying EVs and characterizing the molecular composition of EV preparations are equally important and should include the analysis of both EV-enriched markers and contaminants. While many established analytical methods can be applied to the EV field, novel tools that fit more specific needs are also in development.
Particle level
There are several methods to assess EV preparations on a particle level, which usually includes quantity, size and morphology. One of the oldest methods to characterize particles in suspension is dynamic light scattering (DLS), which relies on illuminating samples with a monochromatic light source and analyzing light scattered by particles to calculate their size. While still widely used, DLS only captures bulk signal from all particles and therefore struggles with polydisperse samples. This limitation was partly overcome by Nanoparticle Tracking Analysis (NTA) techniques, which also record scattered light but track each particle individually. Based on the Brownian motion reflected in scatter patterns, particle sizes can be calculated using the Stokes-Einstein equation. NTA is therefore capable of both counting particles and providing more granular size distributions than DLS. One of the biggest challenges of NTA is that current platforms are unable to distinguish between EVs and non-EV particles of similar size. As EV preparations are commonly contaminated with protein aggregates, lipoproteins and other nanoparticles, NTA-based analysis of low-purity samples is bound to overestimate EV concentrations [7]. Fluorescence-enabled NTA, which tracks scattered laser light at a specific emission wavelength, was recently developed to overcome this shortcoming. Using lipophilic dyes or fluorochrome-conjugated antibodies, NTA’s specificity can be narrowed to particles surrounded by lipid membranes or decorated with marker proteins, respectively [8, 9]. Despite still facing technical challenges, this approach has enormous potential upsides and could significantly enhance detection of EVs and individual subpopulations. An alternative, non-optical, method is Tunable Resistive Pulse Sensing (TRPS), in which EVs flow through a nanopore, and changes in ionic current are used to compute particle sizes and concentration.
Flow cytometry (FCM) has emerged as another method that allows the detection and characterization of thousands of EVs in suspension. However, most conventional flow cytometers are not yet capable of detecting EVs with diameters of less than 300 nm due to limited sensitivity and resolution. To overcome this obstacle, bead-based (e.g. beads coated with antibodies against EV surface proteins) procedures were designed to artificially enrich EVs and increase their fluorescent signal. In addition, bead-free protocols that take advantage of fluorescent-labeling strategies were established to perform multiparametric characterization of EVs and to assess EV heterogeneity. Double labeling with protein- and lipid-specific dyes also enables the discrimination of EVs and common contaminants. However, technological progress accelerated the development of next-generation flow cytometers that allow label-free analysis of EV sizes and concentrations via the scatter channel. By combining the capabilities of flow cytometry with high resolution imaging at the single-particle level, imaging flow cytometry (IFCM) facilitates precise EV characterization and phenotyping [10, 11].
Microscopy-based techniques are frequently used to visualize the morphology of single EVs. Accurate EV imaging can uncover the shapes, structure and size of vesicles as well as other non-vesicular components [12]. Although there are various imaging technologies, resolution limits of each method have to be considered. As a result of sample preparation preceding EV imaging, not all visualization tools can reveal the natural EV morphology. The most prominent visualization technique is electron microscopy (EM). Transmission electron microscopy (TEM) is a standard imaging method that provides structural information about EVs and background contamination in EV preparations. TEM analysis requires extensive sample preparation, which can lead to dehydration of EVs and morphological artefacts (e.g. cup-shaped appearance of vesicles). Cryo-electron microscopy (cyro-EM) allows preserving the native spherical shape since the EV samples remain in their native hydrated state. Immunogold labeling combined with EM analysis (immuno-EM) is a powerful labeling strategy to localize specific proteins in EV preparations. In immuno-EM, gold probes serve as reporter molecules that are conjugated to specific antibodies. Topographic information about EV surfaces can be obtained by scanning electron microscopy (SEM). Atomic force microscopy (AFM) is another method to gather information about the three-dimensional shape (i.e. topography) of isolated EVs. AFM can be performed with vesicles immobilized on distinct surfaces, or operated in liquid, which preserves the physical EV properties. While advanced EV labeling techniques and high-resolution imaging technologies are in development, the molecular composition of EVs remains to be comprehensively characterized.
Analyte | Method | Advantages | Disadvantages |
Particle | DLS | Analysis under physiological conditions | Limited specificity, low resolution of polydisperse samples |
NTA | Improved size resolution, analysis of EV charge | Limited specificity, struggles with fluorescence | |
TEM | Visualizes EV morphology and potential contaminants | Dedicated equipment, toxic chemicals, morphological artifacts due to sample preparation | |
Cryo-EM | No artifacts due to fixation and dehydration,native morphology, stain-free visualization | Dedicated equipment, freezing artifacts, low signal-to-noise ratio, cumbersome sample preparation and handling | |
Immuno-EM | Visualizes EV morphology and specific proteins | Dedicated equipment, cumbersome sample preparation, toxic chemicals, reliance on high-quality antibodies | |
AFM | Works in air, vacuum, and liquids, 3D imaging, convenient sample preparation | Dedicated equipment, data tip-dependent, tip can damage the sample | |
FCM | Rapid assessment of sizes, counts and cluster identification, potential for single-EV analysis, multiparametric, sorting can be implemented | Limited sensitivity and resolution, bead-based approaches are only semi-quantitative, expensive equipment and specialized operators | |
Protein | WB | Low cost, established protocols, simple readout | Low throughput,reliance on specific antibodies, lack of widely applicable EV protein loading controls |
Mass spectrometry | High sensitivity and resolution, quantitative methods are available, identification of PTMs, simultaneous detection of multiple proteins, adjustable to various research questions | Time-consuming, expensive instrumentation, requires very clean sample preparation, high risk of contamination, complex data analysis, sample and setting-dependent limitations | |
ELISA | Established protocols, availability of commercial kits and self-made protocols, direct and indirect detection, simple readout | Immunoreactivity is antibody-dependent, cross-reactivity | |
Nucleic acids | (RT)-(q)PCR dPCR | High sensitivity, relatively cheap, simple workflows, established protocols | A priori knowledge is required, expensive (dPCR), lack of widely applicable EV reference sequences |
NGS | High throughput, de novo detection of novel transcripts, high resolution, detection of sequence variants | Expensive, laborious sample preparation, dedicated equipment, complex data analysis | |
Northern/Southern blot | High specificity, identification of RNA/DNA sequence lengths and alternative splicing products | Lower sensitivity compared to newer methods (e.g. PCR), requires large amounts of sample, limited number of sequences can be analyzed in parallel, time-consuming, toxic chemicals | |
Microarray | Established protocols and analysis pipelines, simultaneous analysis of multiple genes | Restricted to pre-defined sequences, hybridization-dependent, low signal-to-noise ratio | |
AFM: atomic force microscopy; DLS: dynamic light scattering; dPCR: digital PCR; ELISA: enzyme-linked immunosorbent assay; FCM: flow cytometry; NTA: Nanoparticle Tracking Analysis; NGS: Next-Generation Sequencing; qPCR: quantitative polymerase chain reaction; PTM: post translational modification; TEM: transmission electron microscopy; WB: Western blot |
Table 2: Comparative overview of commonly used EV characterization methods.
Molecular level
Many previously established techniques have been used to characterize EVs on a molecular level and revealed the presence of common EV cargo molecules present in almost all isolated EVs as well as tissue-specific signatures present in EVs derived from distinct tissues [13]. Particular interest has been paid to vesicular proteins, which can be used to both substantiate EVs and assess levels of contamination.
Over the past three decades, thousands of proteins have been found in and on the surface of EVs. Deciphering the EV protein composition is interesting for three reasons. First, proteomic studies on EVs provide clues about the molecular mechanisms involved in EV biogenesis and cargo sorting. Second, membrane-bound and cytosolic proteins present or enriched in almost all EVs or specific EV subtypes, as well as proteins not expected to be present in EVs, are identified. It is therefore recommended to analyzed vesicle preparations to demonstrate the presence of EVs and evaluate the depletion of common contaminants [14]. Third, large-scale proteomics datasets suggest EV protein biomarkers for certain diseases that might be relevant from a clinical and biological perspective. Since EVs are usually isolated in small amounts, sensitive methods are required to analyze their protein composition. After protein digestion and sample preparation, EV peptides can be subjected to mass spectrometry, which allows the detection, identification, characterization, and quantification of EV proteins. Additional, mass spectrometry can also be used for the analysis of posttranslational modifications (e.g. phosphorylation, acetylation, glycosylation) and other EV-associated molecules (e.g. lipids and metabolites) [15]. Western blot analysis is another very common approach to analyze the protein composition of EVs. In this method, proteins are separated according to their molecular weight by electrophoresis (e.g. SDS-PAGE) and then transferred onto a membrane to allow antibody-mediated identification of specific proteins. Other antibody-dependent protein methods are for example enzyme-linked immunosorbent assay (ELISA), and immunoprecipitation. Since proteins, particularly lipoproteins, are highly abundant in some biofluids, protein assays should also be used to identify potential contamination in the preparation.
Nucleic acids, including DNA, mRNA and non-coding RNA such as microRNA, are amongst the most frequently studied EV components. Depending on the respective scientific question, experiments might include characterizing complete nucleic acid profiles or quantifying levels of individual species. Facilitated by high-throughput Next-Generation Sequencing (NGS) approaches, DNA and RNA in EVs can be deciphered at single-nucleotide resolution without a priori sequence knowledge. Alternatively, specific sequences that are of interest can be detected and quantified using quantitative PCR (qPCR), RT-qPCR or digital PCR. Combining analytical assays with upstream nuclease and/or proteinase digestion steps is a common way of determining if nucleic acids are genuinely encapsulated in EVs, i.e. protected from nucleases, bound to their surface or associated with non-EV protein carriers [16].
The quest for standardization and reproducibility
The need for standardized EV isolation and characterization methods became painfully clear in 2017, when an international consortium published a meta-analysis of over 1,000 studies from various areas of EV research [17]. Some of the most noteworthy findings of the EV-TRACK report included the heterogeneity of methods used in the EV field – including a total of 1,038 individual EV isolation protocols – and the poor reporting of experimental details in publications. Driven by the need for transparent methods and rigorous EV characterization, EV-TRACK has since morphed into both a coaching tool for the community and an extensive repository of methodological data. Uploading experimental details and reporting the resulting quality score (EV-METRIC) in publications is encouraged to enhance transparency and reproducibility in the quickly growing community. Additionally, EV-TRACK now aggregates biological information on EVs from over 2,000 experiments and is thus a valuable resource in the quest to better understand EV heterogeneity, quality and physiology.
As another important effort to improve EV experiments, the Minimal Information for Studies of Extracellular Vesicles (MISEV) guidelines, was published by the International Society for Extracellular Vesicles (ISEV) in 2014 [18] and updated in 2018 [14]. These guidelines provide practical recommendations for various areas of EV research, ranging from terminology and sample processing to EV characterization and functional studies.
Both the rapid growth of the EV field and the widespread use of high-throughput characterization techniques have led to an accumulation of large amounts of EV profiling data. In addition to established repositories for nucleic acid and protein sequences, there are now dedicated data bases for EV-associated molecules. ExoCarta [19], EVpedia [20] and Vesiclepedia [21] provide searchable information on EV analytes from dozens of species and hundreds of studies.
Conclusion
While presenting intriguing opportunities for both basic biology and translational application, the study of EVs is fraught with obstacles. Despite the abundance of available isolation methods, universally applicable and standardized protocols remain elusive. Each method must be carefully considered regarding its suitability for a given experiment, which depends on various factors including the type and starting volume of biofluid, desired level of purity, downstream assays, experience of operators and the respective scientific questions. Owing to the growing recognition of EV heterogeneity and the need to not only separate EVs from contaminants but also fractionate EV subpopulations, linear combinations of isolation methods are increasingly utilized. Depending on the respective experiment and the required degree of purity, this might mean combining enrichment and purification methods or integrating orthogonal methods that target different EV characteristics. As demonstrated in a variety of research articles, each method is inherently biased towards specific EV populations, which makes thorough characterization of resulting isolates essential for any EV workflow. Using different methods to isolate EVs from the same biological sample was shown to qualitatively and quantitatively alter the composition of resulting vesicle preparations [22-24]. The diversity of EV isolation protocols used by different researchers therefore considerably complicates comparison of the results obtained by individual laboratories, emphasizing the need of standardization. Similarly, a multitude of analytical methods has been used to study EVs on the macro and micro level. Assays based on light scattering, TRPS, flow cytometry and imaging allow characterization of individual particles while high-throughput technologies such as NGS and mass spectrometry significantly contribute to deciphering their molecular composition. Despite the availability of valuable analytical tools, each method brings about its unique limitations, which impedes progress towards more fully understanding vesicle biology. Among other pressing questions, the identification and separation of vesicle subclasses, as well as potentially specific cargo sorting remain two of the most studied areas in the EV field. Even though proteins and nucleic acids share the spotlight in EV profiling studies, we would be remiss not to mention other analytes such as lipids and metabolites that also deserve attention. In addition to the most important methods discussed here, further techniques including super-resolution microscopy, surface plasmon resonance spectroscopy, Raman spectroscopy and single particle interferometric reflectance imaging are successfully used to study EVs. Detection and sensitivity limits of established methods are, however, not always compatible with the EV field’s requirements, which prompted the development of dedicated instrumentation for nanosized vesicles.
Driven by technological advances and a growing understanding of EV biology, the enthusiasm about EVs seems unabated. The past decade of EV research was characterized by explosive growth, and EVs made their way into virtually all areas of life science, ranging from mental disorders to infertility research and ocular diseases. At the same time, it featured a remarkable learning curve for the community, which was confronted with the limitations and challenges of this young and rapidly expanding discipline and in turn quickly implemented measures to boost experimental rigor, increase transparency and, ultimately, enhance the validity of EV studies across the board. While it is too early to tell if the EV-TRACK and MISEV initiatives will leave a lasting impact on the field, their exponential citation rates and the fact that publications citing the guidelines consistently score higher EV-METRICs are promising harbingers of their success [25]. Given a wide adoption of good experimental and reporting practice, EV research is bound to flourish, paving the way for novel insights into basic biology as well as clinical and biotechnological applications.