Extracellular vesicles in developmental biology
Extracellular vesicles – developmental messengers of tissue crosstalk
DOI: https://doi.org/10.47184/tev.2019.01.04During development, EV secretion and the specific loading of signalling factors in EVs contributes to organ development and tissue differentiation. Different biomolecules such as proteins, lipids and nucleic acids transmit these signals and the content, size, and membrane composition of EVs are highly dynamic and depend on the cellular source, state, and environmental conditions.
Keywords: cell-cell communication, exosomes, developmental signaling, morphogen signaling, model organisms
Introduction
In development, multicellular organisms face the challenge of building a body from scratch. This embryonic self-organisation is regulated in space and time and by generation and decay of signals and forces. Cellular communication depends on a common code understood by transmitting and receiving cells and the successful transmission in the presence of noise. Recently, Extracellular vesicles (EV) have emerged as membranous signal carriers that fulfill several of these requirements and represent a cellular communication system that allows individual cells to integrate into an organism and function as information units for an individual cell or a cell population. Studies in different model organisms, i.e. Drosophila, zebrafish or mouse reveal their universal role as signal carriers at the organ or systemic levels during development and homeostasis.
As the contribution of EV to tumour progression, e.g. growth, vascularisation, immune invasion and metastasis is recognised more and more, a deeper insight into their biology in health is crucial. In this review we will focus on different in vivo studies to date that elucidate the function of EV under physiological conditions. In general, we here discriminate three types of signalling principles and compare them to human ways of communication: (1) Transfer of EV to recipient cells, (2) EV-bound signal crosstalk and (3) Systemic role of EV-bound signals.
The telegram – EVs for unidirectional signal transfer
The most direct way of communication is the transmission of information from a donor to one or more recipients. For intercellular communication, this translates to the delivery of a messaging molecule from one cell to another or to multiple receiving cells. In a developmental context, this is commonly employed when signalling molecules are produced and secreted from a distinct population of source cells in order to spread across the extracellular space and induce target cell responses depending on their absolute or relative concentration. Such morphogen gradients govern the patterning and growth of multiple tissues during development of vertebrates and invertebrates and can be used to study how these molecular messages are sent in a manner that is suitable to orchestrate the development of complex tissues and organs. In order to coordinate cell behaviour and growth on a tissue level, morphogen signalling has adapted to encode information on multiple levels and provides highly context-specific information in a space-, time- and dose-dependent manner.
This high degree of regulation requires tight control over morphogen secretion, their extracellular spread and target cell activation. The release of morphogens on extracellular vesicles is one way how cells secure this spatiotemporal regulation and fine-tune signal strength distribution for long-range cell communication. In Drosophila, EV-mediated spread of Wingless (Wg), the main Drosophila Wnt protein, and proteins of the Hedgehog (Hh) morphogen family have been found to contribute to gradient formation across the wing imaginal disc (WID) epithelium during wing development [1]. The lipid-modifications of both proteins render them hydrophobic, a property that limits their (uncontrolled) extracellular dispersion and raises the need for diffusible carriers. Wg was found in distinct punctae colocalising with membranous glycosyl phosphatidylinositol-GFP and the human exosomal marker-construct CD63-GFP in the extracellular space of WIDs [2]. In vitro studies confirmed the presence of signalling active Wg on exosomes from cultured Drosophila S2 cells [3], [4]. Exosome secretion, extracellular levels of Wg and Wg target gene activation were controlled by the SNARE protein Ykt6 [3].
In mammals, secretion and extracellular spread of Wnt proteins on EVs plays an important role during sperm development. Spermatozoa undergo a week-long maturation process that involves changes in protein composition and subcellular localisation and is necessary for their mobility and consequently mouse fertility [5]. This crucial maturation step occurs during their transport along the epididymis, a coiled tubular system that connects the testis and the vas deferens. In mice, this process heavily relies on post-transcriptional Wnt signalling [6]. In order to deliver the required Wnt signal, Wnt ligands are packaged into exosomes and secreted from epididymal cells into the epididymal lumen [6]. These Wnt-bearing exosomes in the luminal fluid are signalling active and induce WNT/STOP signalling in the transiting spermatozoa population and thus promote sperm maturation and fertility. Here as well, EVs are used as a shuttle for the delivery of a required developmental stimulus to a target cell population. It is not clear yet, whether EV are merely the carrier for hydrophobic Wnts or whether additional EV signals, such as RNAs, are contributing to an overall differentiation programme transferred by EVs.
Similarly to Wg, Hh is secreted on EVs from its Drosophila WID source cells, where it colocalises with CD63-GFP as well as MVB markers [7], [8]. Interference with ESCRT-mediated ILV formation and EV secretion impaired extracellular Hh transport and long-range target gene induction [8], [9]. Similarly to Wg, in vitro EV-bound Hh only contributes a fraction of the overall signalling capacity [8].
The long-range transport of Wg and Hh is especially interesting, as both morphogens undergo post-translational lipidation that negatively affects their solubility and hence their ability for free diffusion [1]. Packaging these morphogens on vesicle carriers however does not only enhance their signal range by increasing their extracellular mobility, it also adds additional levels of regulatory control over their extracellular amounts by allowing context-dependent endosomal routing, sorting and secretion. In line with this idea, Hh-containing MVBs and EVs have been shown to travel along cytonemes, dynamic signalling filopodia that spread out from the basal side of source cells, to even further increase the local and temporal precision of Hh delivery to its dedicated target cells [7], [10]. Although a similar mechanism has been proposed for Wg signal transduction, cytoneme-based secretory Wg transport in WID has not yet been demonstrated. In addition to EVs, also lipoprotein particles have been described as vehicles for long-range signal transduction of both Wg and Hh in WID [11]. This apparent diversity of extracellular trafficking routes indicates that secretory cargo shuttling is highly context dependent and highlights how much energy cells invest in order to gain control over extracellular morphogen levels and their precise timely and spatial delivery. The secretion of Hh and Wg from their source cells on EVs and their subsequent spread across the extracellular space for the delivery to target cells represents a prime example of how EVs can act as carriers for the unidirectional transfer of signalling molecules. While this one-sided molecule transfer is sufficient for the guidance of many developmental processes, more complex developmental challenges require reciprocal exchange of information. Many examples exist in which EVs act as vehicles for the exchange of information between one cell group and another cell population.
The phone call – EVs for reciprocal intercellular communication
For development of complex tissue structures, cells of different origin and determination need to exchange signalling information with one another in order to coordinate the joint development into tissues or organs. Bidirectional exchange of signalling molecules can be mediated by secretion of EVs and their reciprocal uptake by the respective other cell population. Such EV-bound crosstalk occurs between the surface epithelium and underlying mesenchyme during organogenesis and is necessary for the coordinated development of different organs, such as skin, mammary glands, lung and kidney.
During tooth development, EVs secreted from both epithelium and mesenchyme cells diffuse through the basement membrane and are then preferentially taken up by cells of the respective other tissue type. After uptake they reciprocally induce cell differentiation and matrix synthesis: miRNAs transferred by epithelial EVs stimulate mesenchyme cells to undergo mineralisation while mesenchymal EVs induce the production of basement membrane compounds in epithelial cells [12]. But EV transport much more than single signalling molecules – they transfer a detailed description of the sending cells’ content. This integration of context-dependent developmental requirements allows cells to adjust to changing environmental needs and is necessary to coordinate their growth into functional tissues.
To facilitate directed EV exchange between non-adjacent cells, cell protrusions or filopodia have been found to function as signalling highways, enabling directed bidirectional cargo transport to and from the corresponding signalling partner. During early Xenopus development, the specific distribution of morphogens is necessary for the induction of a first dorso-anterior specific gene expression pattern. This process is guided by the precise localisation and reception of maternal morphogens during blastocoel expansion and makes use of filopodia spread from the basolateral side of the Xenopus blastocoel to connect blastomeres over multiple cell diameters [13]. EVs are shed from the tips of such filopodia to allow active delivery of morphogens, receptors and cytoplasmic proteins to surrounding blastomeres. At the same time, EVs are actively taken up at the filopodia bases themselves, turning them into signalling hubs that facilitate an active exchange of proteins and cytoplasmic content [13].
Other examples for reciprocal communication are found in the nervous systems, when neurons and glia cells interact during neural circuit development and synaptic plasticity. In response to neuronal signals oligodendrocytes secrete exosomes. These enter neurons to make their cargo functionally available to the neuronal metabolism and improve axonal growth and survival [14]. This also applies to regenerative capacities of neurons in the CNS, that are improved by fibroblast–derived exosomes inducing Wnt signalling [15]. Moreover, synapse maintenance and plasticity is regulated by the exchange of signals between neighboring neurons. The release of the transmembrane protein PRR7 on exosomes in an activity-dependent manner and the uptake of those exosomes into neighboring neurons reduces excitatory synapse numbers by blocking exosomal Wnt secretion and signalling in the recipient cells [16].
The twitter message – EVs for systemic communication
EVs can be found in virtually any body fluid, ranging from blood plasma over urine to even breast milk [17] . Hence, signalling information in the form of proteins, lipids and nucleic acids carried on EVs can be distributed systemically throughout the whole body and is available to an enormous audience of potential target cells. At the same time, EV-based communication is eligible to any cell type that contacts extracellular space and blood stream and basically all cell types and tissues have been found to secrete EVs [17]. The resulting heterogeneity of vesicles of individual origin, cargo and purpose travelling in the blood stream is overwhelming. This sheer amount of information and signalling complexity turns understanding the function and biology of individual EVs into a challenge. However, in vivo developmental model systems can provide means to study systemic EV-mediated intercellular communication, as they allow the direct visualisation and live imaging of EV secretion, travel and uptake. For example, translucent zebrafish embryos can be used to visualize fluorescently labelled exosomes in vivo. Especially the expression of a CD63-pHlourin construct that links the exosomal marker CD63 with pH-dependent GFP has proven useful to visualize EV secretion events from single cells [18]. Expression of a CD63-pHlourin reporter in zebrafish embryos allows the tracking of individual EVs from their site of secretion at the yolk syncytial layer throughout the blood stream until uptake into their target cells, macrophages and epithelial cells at the caudal vein plexus [19]. Interference with exosome biogenesis in the syncytial yolk sac affected growth of the caudal vein plexus, underlining the biological relevance of this systemic EV transfer. In addition to contributing to our understanding of EV biogenesis and function during development, such in vivo model systems are used to study the secretion and fate of EVs during disease conditions. Using a zebrafish melanoma model, it was possible to track tumour-derived EVs throughout the zebrafish embryo and identify patrolling macrophages and endothelial cells as their major recipient cell population. EV uptake and subsequent activation of macrophages was furthermore found to stimulate metastatic outgrowth, demonstrating the functional relevance of systemic cross-tissue EV exchange for cancer progression [20].
Another example for a systemic role of EVs was recently discovered in the immune system of Drosophila fruit flies. In invertebrates, antiviral immunity is based on a nucleic-acid-based, sequence-specific mechanism (RNA interference). Macrophage-like circulating immune cells, called haemocytes, amplify and spread antiviral signals by taking up viral RNA from infected cells and copy viral cDNA templates to generate small viral RNAs. These vsRNAs are packaged and secreted into EVs. Systemic spread of those conveys antiviral activity and virus-specific immunity for all up-taking cells. This immunity lasted several weeks after clearance of the virus and therefore represents an adaptive immune system not previously anticipated in flies [21].
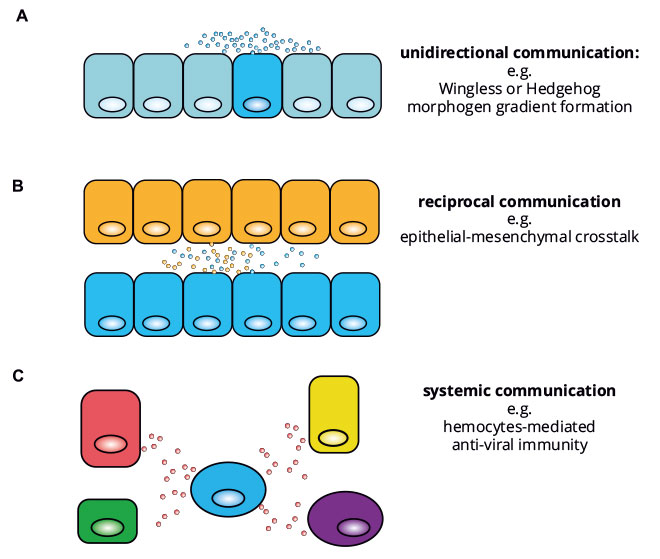
Conclusion
As demonstrated by these examples, EVs play an important role in tissue crosstalk under physiological conditions. The context specificity of EVs, the signals exchanged and induced changes in cellular behaviours are beginning to emerge from these in vivo studies. In the way they package and combine information from multiple sources, and similar to viruses, EV seem to function as small programs that are sent out to coordinate and modulate cell behavior at a tissue or organismal level. In light of the potential clinical applications of these messengers, these and further in vivo model systems should be the focus of more detailed studies in development and homeostasis.