Extracellular vesicles flow cytometry analysis
Analysis of extracellular vesicles by flow cytometry – basics, limitations and prospects
DOI: https://doi.org/10.47184/tev.2019.01.06Flow cytometry is a well-established technique that is classically used to detect cells and quantify related parameters on the cellular surface, e. g. the expression of surface protein markers. Within the last few years, there also have been considerable advances of using flow cytometry to detect and quantify extracellular vesicles.
Keywords: Flow cytometry, Extracellular vesicle, Surface markers, Exosome, Microvesicle
The essential basics of flow cytometry
For a better understanding we first want to illustrate the principles of flow cytometry (FCM). Flow cytometry has evolved over the last few decades and nowadays is a well-established technique that determines events based on their physical and/or chemical characteristics. Samples containing single events (classically cells) are injected into a flow cytometer and pass one or more laser beams in a fluid stream. The resulting light signals are then detected, quantified and displayed in a software after appropriate processing. Typically, depending on the type of flow cytometer used, between 10,000 and 40,000 events per second can be analyzed.
Classically, flow cytometry is used to measure cells and analyze different parameters such as surface protein expression, cell cycle or viability, but within recent years instruments and methods have been developed which also allow for the detection of much smaller particles such as extracellular vesicles (EVs) or viruses [reviewed in [1, 2]]. Flow cytometry was initially developed in the 1960s and especially after fluorochrome-conjugated antibodies have become available, it has been used for numerous applications for basic biological and especially immunological and hematological research. Three decades later, it reached clinical breakthrough with HIV and leukemia/lymphoma diagnostics becoming mainstream. Today, flow cytometry has become indispensable in research and clinical diagnostics and it is one of the most impressive and versatile techniques for characterizing single cells in a high-throughput, multi-parametric manner [reviewed in [3]].
For the sake of simplicity and for better understanding, we here first describe the basic principle of the technique of flow cytometry on cells [4]. As we will see later, the preliminary preparative and analytical methods suitable for analyzing extracellular vesicles are fundamentally different from that used for cells. The light signals generated by cells passing lasers lead to light scattering around the cell and also to the excitation of fluorescent dyes (fluorochromes), which then emit a corresponding light signal. As already mentioned, flow cytometric analyses of cells can be performed to collect a variety of different information, the most prevailing information being if a surface protein is present on the cellular surface or not, by using a fluorochrome-conjugated antibody specifically raised against that surface protein. Furthermore, cells can be also classified based on differences of how they scatter light. Blood cells, for example, can roughly be divided into three basic groups (lymphocytes, monocytes, and granulocytes) merely based on how they scatter light, since these cell types have different sizes and a different granularity (Fig. 1A).
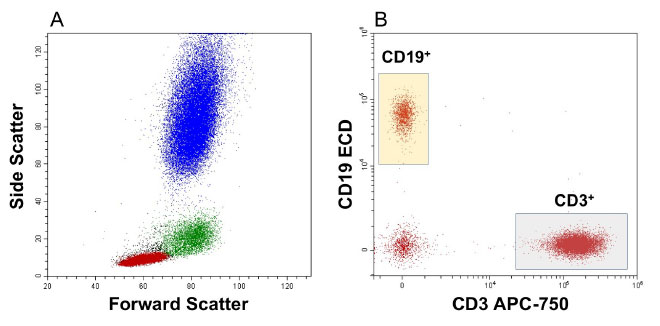
If these cells are then additionally labelled with antibodies which are directed against certain proteins on the cellular surface, it becomes possible to further identify subtypes within those groups, such as B cells (CD19 positive) versus T cells (CD3 positive) within the lymphocyte population (Fig. 1B). The prerequisite is that the antibodies used for this are conjugated with different fluorochromes which can be distinguished by the instrument used. Overall, the determination of various cell populations is facilitated through the usage of different fluorochromes, which give different light properties after laser light excitation. Thus, in our example, if antibodies recognizing a surface protein specifically expressed on T lymphocytes (CD3) are labelled with a fluorochrome with distinct spectral properties than antibodies being used to recognize a B cell-specific surface marker (CD19), both cell populations can be accurately identified and quantified in the same sample (Fig. 1B).
The use of lasers of different wavelengths and the availability of a very large number of fluorochromes have increased the combinatorial options being available to label and identify different cellular subsets within one sample over time. Nowadays, 30 or even more parameters can be captured simultaneously from one cell with more advanced instruments allowing such high-dimensional analyses [5].
A brief introduction into EV flow cytometry
As mentioned above, flow cytometry can be performed in a relatively simple way with cells. However, it becomes much more difficult when EVs are sought to be analyzed due to various reasons. Cells can be as small as 2-4 micrometers (such as platelets), and they also can have diameters of 20 µm (e.g. monocytes) or even be bigger. Extracellular vesicles, however, are much smaller and subsequently much more challenging to analyze by flow cytometry. The diameter of an exosome-sized EV (~100 nm) is about 100-fold smaller than a 10 µm-sized leukocyte (Fig. 2).
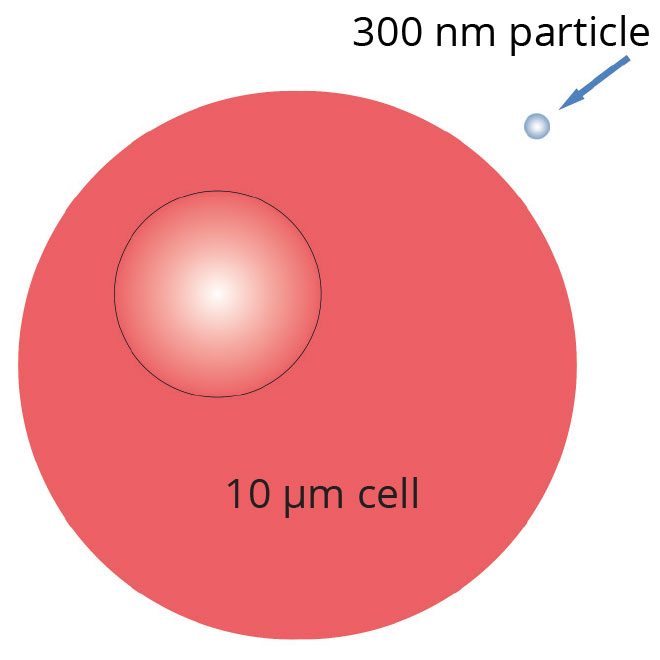
This means that the surface area of a small EV is about 10,000 times smaller and its volume approximately 1 million times smaller than that of a typical cell [6]. As already mentioned, flow cytometry “only” detects the light properties of events, and thus it is clear that very small particles with diameters beyond the wavelength of visible light (~380-700 nm) are very challenging to detect [7]. Those events scatter only very small amounts of light compared to cells, and only few antibodies can bind to a single EV, meaning that that the sensitivity of the method required to detect such small amounts of light will require methods or instruments with very high sensitivity. It is therefore also understandable that older, conventional flow cytometers, which have lower photosensitivity due to their hardware specifications, can only recognize significantly fewer extracellular vesicles with a bias towards brighter or bigger events than newer devices that are significantly more sensitive. A comparison between various conventional flow cytometers published in 2018 demonstrated that only 21 out of 46 flow cytometers tested could detect EVs in a range between 600 - 1,200 nm and not smaller [8]. Therefore only few instrument are sensitive enough to detect particles below sizes of around 300 nm [9] and thus would only be suitable to analyze a subfraction of the submicron particles in a given sample. Consequently smaller events would be just missed and would not be detected above background levels in that case. In worst case, one would just detect the ‘tip of the iceberg’ and miss all other EVs that are just below the limit of detection when using conventional and rather insensitive instruments.
Sometimes EVs can come in swarms
Another critical factor is the design type of the flow cytometer and the inherent increased likeliness of EVs ‘coming in swarms’ which can lead to data misinterpretation as you will read below. Depending on the flow cytometer being used, the generated laser beam can have a height of up to a few micrometers. If cells of a size of about 10 µm pass the laser beam, it is relatively likely that only one cell passes the laser beam during laser excitation, and thus will be recognized as a single cell. For EVs this is much different. They have a size that is far below the height of the laser beam. Consequently, several particles or EVs can pass the laser beam at the same time especially when the sample is relatively high concentrated, but the computer does not recognize the incoming signal as a signal of several particles, but instead interprets the signal as one single ‘event’. This effect also called coincidence and in severe cases ‘swarm effect’ [10, 11]. The swarm effect is thus not only responsible for not correctly reproducing the exact concentration of EVs per unit volume, but also for detecting signals received from individual EVs. Several events with different fluorescence characteristics are not detected as individual events and therefore fluorescence signals of all particles measured as one event are cumulatively quantified, resulting in higher fluorescence intensities. Thus, suddenly particles in a sample containing distinct populations of EVs with exclusive marker expression can be detected as double positive events which actually do not exist. However, coincidence or swarm detection can be avoided by relatively simple means, namely by measuring unknown samples in serial dilutions. If the dilution factors lead to the expected reduced number of measured EVs, the risk for coincidence or swarm detection is minimized and one can make sure that samples are analyzed while being in a linear range of signal detection for the respective sample type (Fig. 3).
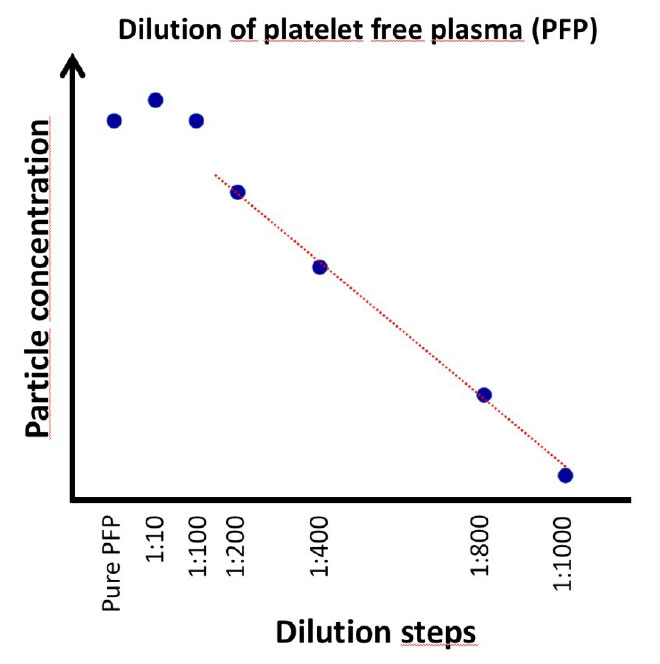
Thus, samples should always be measured at the lowest concentration possible. In addition, to avoid coincident events and to improve instrument sensitivity, EV measurements should generally be performed at the lowest possible measurement/flow speed. Higher measurement speeds often also increase the diameter of the core stream or sample stream which may increase the likeliness of coincidence detection to happen [10]. When using imaging flow cytometry (IFCM) for EV analysis different options how to process the images and quantify the number of distinguishable dots per event/image allows for additional accuracy to quantify coincidence or only include true single events in the EV quantification [9, 11].
Higher sensitivity also
comes with higher background
Another source of potential errors that can lead to data misinterpretation can come from fluorescently labelled antibodies when using them to detect EV surface markers. When staining cells with antibodies, it’s relatively easy to detect signals since in most cases quite some antigens of a certain surface protein are available on the surface of one cell, and it’s relatively easy to wash away unbound antibodies by slow-speed centrifugation. Also, protocols for measurement of cells make it quite easy to exclude small particles or ‘background’ from the analysis just by setting a region to define the main cell population, or a threshold. When antibodies, however, are sought to be used to detect surface markers on EVs, this can lead to certain limitations. First of all, the composition of surface proteins present on the surface of EVs often resembles the surface protein signature of the cells releasing them. For example, EVs derived from platelets carry platelet-related surface markers such as CD41, and EVs released from red blood cells also carry erythrocyte-specific surface markers such as CD235a or GlycophorinA (Fig. 4). However, because the surface of EVs is significantly smaller than the surface of cells, significantly fewer antibodies can be bound to the surface of a single vesicle. This, in turn, means that the fluorescence detection of the instrument used has to be sensitive enough to detect the signals of only few fluorochromes, or relatively few antibodies bound to a single EV. As mentioned before, several commercially available flow cytometers are sensitive enough to achieve this [9, 12-15].
However, this increased sensitivity and therefore the really low detection limit leads to another potential pitfall for such antibody-based assays: Commercially available fluorescence-conjugated antibodies presently are not validated for EV flow cytometry, and different groups have reported that the usage of such antibodies can lead to a considerable amount of background which is probably related to protein complexes or antibody aggregates [9, 16, 17]. To account for this, it is therefore essentially required to include so-called ‘buffer controls’ containing the antibodies in PBS at the same concentrations as in the sample of interest (but without any EVs), and it is also strongly recommended to centrifuge or filter antibody solutions before usage to reduce this kind of background. Other recommendations for example comprise a detergent lysis control to demonstrate that actual membranous vesicles are measured [9, 18-20].
Does size matter?
The brief answer to this question is definitely yes. Firstly, differences in size can relate to different EV subsets with distinct functions, e.g. small EVs like exosomes or small microvesicles might be more relevant for a therapeutic or diagnostic approach than bigger EVs. Secondly, knowing the accurate size of EVs in a sample or the size range a certain method can measure can be very important to make appropriate conclusions and to compare data between labs or instruments. EV size nowadays in most cases is measured by a method called Nanoparticle Tracking Analysis (NTA) which is based on the detection of scattered light of particles and their movement in solution [21]. In the last decade, there also have been several studies using polystyrene or silicate beads to set size gates for EV flow cytometry experiments [22]. Meanwhile it is well known in the field that a proper size determination by simply using such beads as reference material is not valid since the refractive index, which describes the light scattering properties of a particle, drastically differs depending on the material and thus is very much different between a polystyrene (PS) bead and a biological vesicle. For example, Chandler et al. demonstrated that – in terms of their light scattering properties - 0.4 µm PS beads reflect EVs with a size >1µm [23]. Therefore, currently available beads have a refractive index which, depending on the type of beads used, is more or less far away from the refractive index of biological material, making accurate sizing almost impossible. Of note, two recent studies have described how measured scatter signals can be converted to absolute size by using appropriate scatter calibration standards and modelling based on Mie theory [13, 24]. Another group recently showed that viruses might represent another promising biological reference material for EV size calibration [22]. Taken together, these works demonstrate that it is generally possible to combine multiparameter fluorescence detection with absolute sizing for single EVs by flow cytometry, which overall underlines the promising potential of EV flow cytometry.
In addition, calibration of fluorescence intensities also has come up relatively recently in the EV field and is required to achieve a generally great comparability between results from different labs or generated with different methods or instruments [9, 14]. In relation to that, it has become clear that the field highly desires more suitable or improved reference and calibration materials such as beads made from more EV-like materials or biological reference materials [9, 25, 26], since most materials being used nowadays were designed and produced for cell-based flow cytometry assays. In a recent publication for example, we have used GFP-tagged EVs as biological reference material to optimize a method called imaging flow cytometry for the accurate analysis of single fluorescent EVs [9]. Nowadays, there is consensus in the field that EV flow experiments require detailed reporting of all steps and parameters, calibrated instruments, and the inclusion of all recommended controls.
Pitfalls and limitations all over? Still, it’s worth it!
Despite all the aforementioned pitfalls and limitations, and despite all required controls, validations and calibrations, why would someone aim to use flow cytometry for EV analysis? The answer is relatively simple: Because the prospects, promises and the potential of EV flow cytometry are worth it. The most important advantage of flow cytometry lies in high throughput single EV analysis and in the possibility of simultaneous detection of different antigens on the surface of thousands of single particles in a relatively short time. In contrast, other methods often only offer bulk analysis or are not very quantitative. Thus, EV FCM makes it possible to rapidly detect co-expressing markers and quantify both the frequency of such an EV population volumetrically and their brightness in terms of absolute units of fluorescence. For illustration, the following approach has been adopted as a standard method in the core facility in Vienna: Some of the cell membrane-derived EVs are endowed with a protein on the cell surface called phosphatidylserine (PS) [27]. PS can be detected in relatively simple ways by flow cytometry by using fluorescent probes. However, as already mentioned, the surface of EVs is relatively small and enough expressed fluorescence labelled PS molecules must be excited, so that an appropriately measurable signal occurs. If this signal exceeds a certain threshold and thus lies within the detection range, this signal is used as a primary input signal for the detection of all other bound antibodies (Fig. 4).
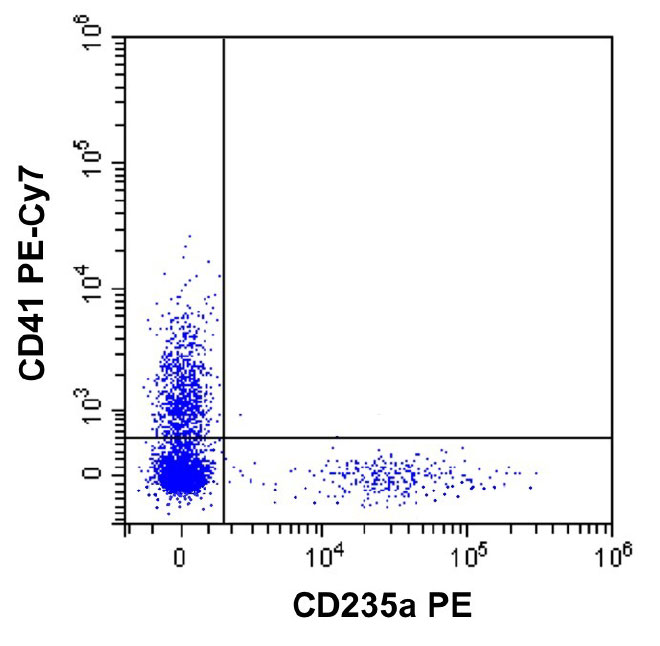
But even with this standardized method we have to compromise for now. On the one hand we need the mentioned signal above the detection limit for the method and instrument we use, on the other hand we use this method to capture only a part of the EVs. Based on previous reports, about 70% of all EVs do not carry PS on their surface and therefore cannot be detected by the method described as such [27]. Obviously, these PS negative EVs can be just as important and should be included in this analysis ideally. Ongoing approaches attempt to include these PS-negative EVs by using dyes that are either incorporated into the particle membrane or absorbed by the particles. The absorbed, initially non-fluorescent substances are then converted into a fluorescent dye via active enzymatic processes within the vesicle. Unfortunately there is no “universal” dye for EV staining available yet and therefore also the usage of such dyes has limitations. However regardless of the limitations, the combination of such dyes and the PS might allow the determination of a larger amount of particles. This example demonstrates that generally assays can already be used even despite limitations, but there are always ideas and potential solutions to improve them.
Conclusion
In summary, several studies using high-resolution flow cytometers have demonstrated proof-of-concept that flow cytometry facilitates the analysis of subsets of extracellular vesicles also down to the size-range of exosomes [9, 12, 14, 15], and also the separation of subsets by flow cytometry based sorting [12, 15] within recent years. The development of more suitable instruments with more sensitive detectors, lower background noise and brighter, more clean and more specific probes will surely further improve such methods in the near future. Despite these advances for flow cytometry based single EV analysis methods, a valid alternative for labs currently lacking access to suitable high resolution instruments could be bead-based flow cytometry assays which are suitable to collect information about the surface markers being expressed on bulk EVs of interest [28, 29]. Also, next generation reference materials including biological reference materials [9, 25, 30, 31] and refined methods for instrument calibration [9, 13, 24] will collectively contribute to the development of standardized assays which ultimately also will suit the requirement for clinical or diagnostic procedures to detect relevant EV based biomarker for various diseases. Finally, several international societies publish both position papers and framework papers, which are regularly updated and can alert interested people to the ongoing process of EV research [18, 19].