EVs in the retina
Shining light on extracellular vesicles
DOI: https://doi.org/10.47184/tev.2023.01.05The present review presents the current knowledge on extracellular vesicle biology in the retina, a highly structured part of the central nervous system that is at the same time easily accessible. Numbers of publications in the field are rapidly rising, but most studies focus on extracellular vesicles as biomarkers for retinal diseases or as putative therapeutic targets. However, rather little has been done so far to elucidate the physiological functions of EVs in the retina. Even though it is clear that all the characteristic functions of EVs such as cell-cell communication, waste removal, extracellular matrix turnover, immune modulation etc. are of extreme importance in retinal tissue with its enormous metabolic turn over and the need to orchestrate broad adaptation to different conditions such as light and dark with as little disturbance of the light path as possible.
Keywords: retina, RPE, Müller glia cells, photoreceptors, extracellular vesicles
Introduction to the retina
Sight is the dominant sense in humans, and the ability to see has a great impact on leading a socially and economically independent life. The dioptric apparatus of the eye focuses light from the surrounding onto the light-sensitive retina in the back of the eyeball. The retina consists of the retinal pigment epithelium (RPE) and the neuroretina. The retina is part of the central nervous system, having derived from invaginations of the anterior neuroectoderm during embryogenesis [1]. It is specialized to convert light into electrical signals that are transmitted to the brain via the optic nerve. The tissue is distinctly structured into three nuclear layers separated by two synaptic (plexiform) layers (Fig. 1).
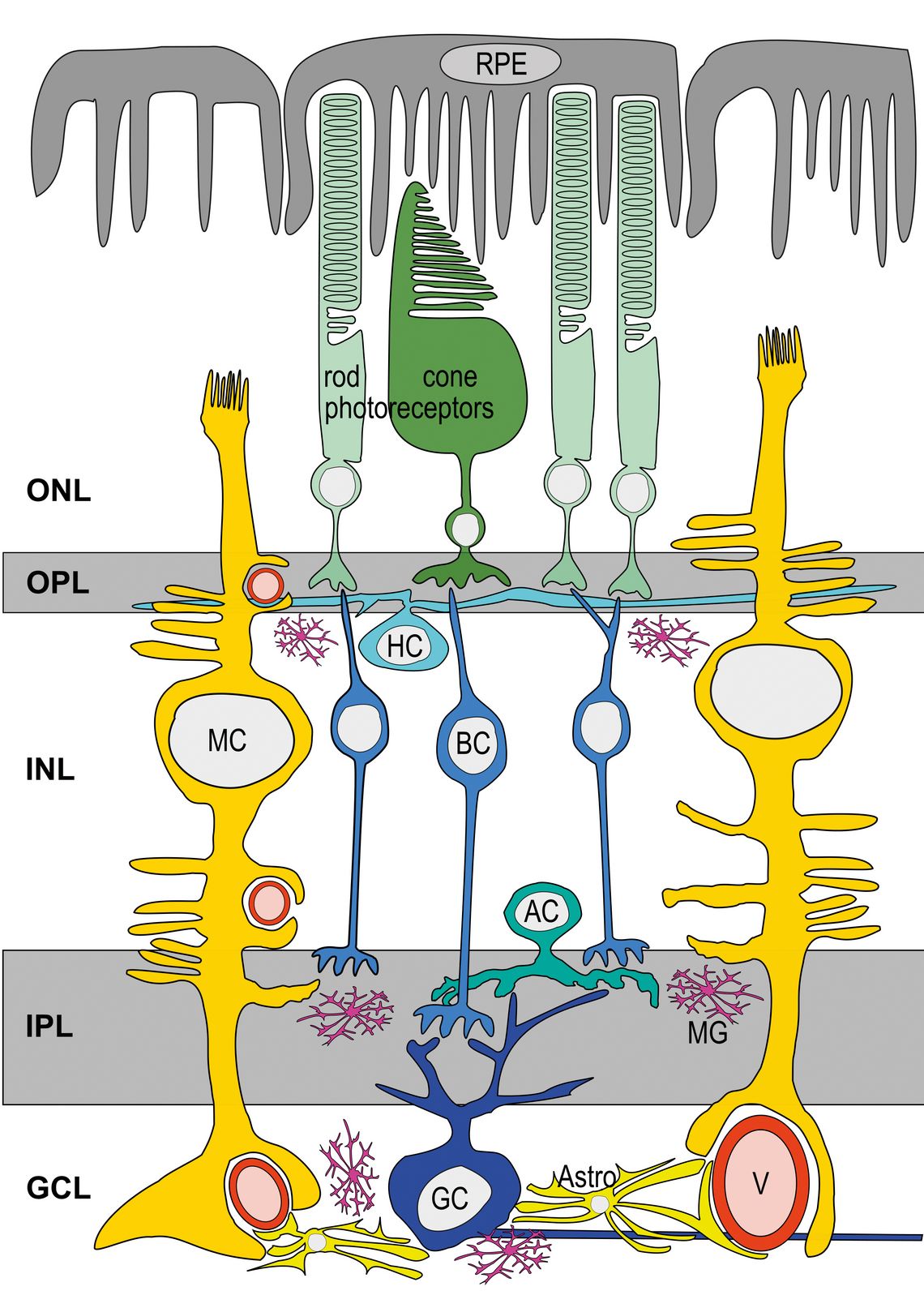
Figure 1: Schematic overview of the retina
The retina beneath the retinal pigment epithelium (RPE, grey) consists of three nuclear layers (outer nuclear layer, ONL; inner nuclear layer, INL; ganglion cell layer, GCL) separated by two synaptic layers (outer plexiform layer, OPL; inner plexiform layer, IPL). The rod and cone photoreceptors (light and dark green) contact bipolar cells (BC, light blue) that, in turn, make contacts with ganglion cells
(GC, dark blue). Horizontal cells (HC, bright turquois) and amacrine cells (AC, dark turquois) modulate the signal. Large radial glia cells, the Müller cells (MC, dark yellow) connect each and every other cell type in the retina, including astrocytes (astro, bright yellow), microglia (MG, pink) and the vasculature (V, red).
The light-sensitive rod and cone photoreceptors are situated in the outermost layer of the neuroretina. The photoreceptors transduce light into electrical signals. Signal integration happens already at the level of the retina by second and third order neurons, namely the bipolar and the ganglion cells, the axons of which eventually build the optic nerve. Horizontal, amacrine and interplexiform cells further modulate the signal [2]. Astrocytes and Müller glia cells, large radial glia that span the whole thickness of the retina, support neuronal function and regulate e.g. the blood flow based on neuronal activity. Microglia, the local tissue macrophages, constantly scan the environment.
All the numerous cell types interact with each other through a wide range of mechanisms. Neurons communicate through classical chemical as well as electrical synapses, but also via ephaptic coupling and mechanisms that modulate the pH of the synaptic region, thereby inhibiting voltage-gated Ca2+-channels important for presynaptic vesicle fusion [3]. The communication between glial cells, the vasculature and neurons is likewise based on intercellular contacts and via the exchange of molecules [4].
Cell-cell communication via extracellular vesicles
More recently, it has become apparent that also extracellular vesicles (EVs) play an important role in the communication between cells of the nervous system [5]. EVs are small (diameter ≈ 30–1,000 nm) membrane-bound particles that may contain proteins, nucleic acids (e.g. mRNA, DNA, and miRNA), lipids, and metabolites from the origin cell [6]. The exact cargo is dependent on the cell type and the physiological or pathophysiological context. Most often, the content as well as the number of released EVs change during cellular stress or disease. Although nomenclature in the literature is inconsistent, EVs are commonly classified into different categories according to their size, biological function and route of synthesis: Exosomes have a diameter of 30–100 nm. They derive from multivesicular bodies through the endosomal pathway and are released to the extracellular medium after fusion with the plasma membrane [7].
Microvesicles (also called microparticles or ectosomes) with a diameter of approximately 100–1,000 nm bud directly outwards from the plasma membrane [8]. With a diameter of 1–5 µm, apoptotic bodies are the largest EVs. They also contain cellular organelles and genetic material [7]. However, despite their distinct nature, the experimental approaches to purify the different EVs are often not sufficient with regards to yield, purity and biochemical composition to fully discriminate between distint EV subtypes or even between EVs and other impurities such as large molecules or cell debris [8]. It is therefore recommended to each time describe the EVs that are studied according to the Minimal Information for Studies of Extracellular Vesicles guidelines “MISEV2018” [9]. Since not every study that is mentioned in this review fulfilled this attempt, the term “EV” is used here even when a more specific nomenclature was applied in the original paper.
Most publications in the field of retina research focus on EVs as biomarkers for retinal diseases or as putative therapeutic targets [10]. However, rather little has been done so far to elucidate the physiological functions of EVs in the retina. Yet, it is clear that all the characteristic functions of EVs such as cell-cell communication, waste removal, extracellular matrix turnover, immune modulation etc. [6] are of ultimate importance in retinal tissue with its enormous metabolic turnover. Additionally, the retina needs to orchestrate broad adaptation to different conditions such as light and dark with as little disturbance of the light path as possible.
EVs secreted by the RPE
So far, research about EVs in the retina has mostly focused on the RPE, a flattened, hexagonal, melanin-pigmented monolayer epithelium firmly attached to the underlying choroid. As part of the blood-retinal barrier, the RPE transports highly selectively nutrients, ions, water and metabolites. The RPE releases many growth factors that are important during development and maintenance of the retina and the choroidal vasculature [11]. On the apical side, the RPE possesses microvilli that envelope the outer half of the outer segments of photoreceptor cells [12]. This close contact ensures that the RPE can fulfill its numerous functions to protect, support and nourish the retina such as recycling of visual pigments and phagocytosis of constantly shed membrane discs from the photoreceptor outer segment [11]. Evidently, pathophysiological changes in RPE cells lead to retinal diseases such as age related macula degeneration [11]. Therefore, the RPE is an attractive therapeutic target. Besides, RPE cells are easy to isolate and maintain in primary cell culture, be derived from induced pluripotent stem cells (iPS-RPE) or embryonic stem cells (ES-RPE), and also artificially (e.g. hTERT RPE1) and spontaneously (e.g. ARPE19) immortalized cell lines exist [13]. ARPE19-cells were found to express EVs that contain Hr44 and CD63 in an inflammation dependent manner [14]. CD63, CD81, LAMP2 and complement factors were also found on EVs [15]. Further studies showed EV release from ARPE19-cells with an effect on immunomodulation [16] or angiogenesis [17-21]. When plated on transwell inserts with a careful differentiation protocol, ARPE19-cells grow in a polarized fashion and secrete EVs containing αB crystallin, a small chaperone, at the apical side rather than on the basolateral side [22]. Interestingly, the inhibition of αB-crystallin expression via shRNA suppressed EV secretion, implying that EV biogenesis or secretion relies upon availability of the respective cargo [23].
However, certain RPE features are lost even in this frequently used cell line [13]. Thus, Klingeborn et al. used mature, polarized primary cultures of porcine RPE cells grown on transwell inserts to characterize the apical vs. basolateral release of EVs by mass spectroscopy [24]. EVs from either side contained proteins fitting to their biological function, e.g. bestrophin-1, a transmembrane chloride channel known to localize at basolateral membrane of RPE, was found on basolaterally released EVs as well as the complement protein C3, the amyloid beta precursor protein (APP) and some extracellular matrix proteins. EVs contaning the transmembrane zinc transporter ZIP12 were apically secreted. The apical release of EVs containing heat shock proteins including αB-crystallin was also confirmed. Apically as well as basolaterally released EVs contained annexin A2 and several integrins. Remarkably, CD9 and CD63 showed a rather low abundance in the basolateral compared to apical EV proteins, providing evidence for distinct EV populations on either side. This fact is of importance for purification methods that rely on EV surface markers.
Highly polarized primary cultures of human fetal RPE were employed to not only confirm the apical secretion of EVs containing αB crystalline, but also their basolateral release under strong oxidative stress conditions [25]. The authors also provide evidence that αB crystalline is subsequently taken up by neighboring RPE cells as well as photoreceptors, providing a protective effect against apoptosis. Interestingly, EVs from stressed ARPE19-cells upon treatment with H2O2 were shown to be taken up by naïve cells through clathrin-dependent endocytosis, whereas EVs from non-stressed ARPE19-cells were not [26]. The uptake was dependent on specific proteins on the EV surface, such as integrins, proteoglycans, and annexin A2, which had higher levels on stress EVs than non-stress EVs.
EVs secreted by photoreceptors (/retinal progenitor cells)
The close, synergistic interaction between RPE and photoreceptors is extremely important for retinal function. In fact, the two cell types act as a functional entity in many ways [11]. As mentioned above, the RPE phagocytoses one tenth of the photoreceptor outer segment discs on a daily basis [27]. The photoreceptor outer segments carry stacks of membrane discs with high amounts of incorporated components of the phototransduction cascade, especially rhodopsin. The outer segment is tied to the metabolically highly active inner segment by a thin structure called connecting cilium. The outer segment is a highly modified primary cilium, the signaling organelle of a cell [28]. Primary cilia are known to shed EVs from their tip by a mechanism called ectocytosis [29]. Indeed, EVs carrying rhodopsin were released by flatmounted murine retinal cultures [30, 31] Mouse retinal progenitor cells release EVs with enclosed miRNA, mRNA and proteins involved in multipotency and differentiation that reflect their expression states [32].
Several studies have shown that rod photoreceptors expel rhodopsin-containing EVs into the intercellular space when correct rhodopsin transport and localization to the outer segment is impaired [33-36]. These EVs are potentially taken up by microvilli of the RPE and degraded [37]. Interestingly, healthy photoreceptor cells release far less EVs and also other types of photoreceptor degeneration do not seem to increase EV production [33]. Mislocalized rhodopsin is toxic to the photoreceptor [34], especially in its activated form [38]. Signal regulation by disposing GPCRs through ectocytosis is a known process in cilia, both, under healthy and pathological conditions [39, 40]. It thus seems that EVs are actively being used to dispose excessive rhodopsin that either accumulated in the inner segment membrane due to the (mutant) lack of an outer segment target motif in rhodopsin [34] or a defective transport machinery within the cell [33].
Also in the rds-/- mouse, an established model for photoreceptor degeneration, which lacks the structural protein peripherin 2, very high amounts of EVs accumulate in the interphotoreceptor space, while no outer segments are formed [41]. However, material disposal does not seem to be the main reason for that. Rather, an erroneous outer segment disc neogenesis [42].
The fact that 10 % of the outer segment is phagocytosed every day implies that an enormous amount of proteins and lipids needs to be shuffled from their place of synthesis, namely the inner segment, towards the outer segment to build up fresh stacks of membranes. It is known that intracellular transport along microtubules brings vesicles with outer segment material like rhodopsin towards the base of the connecting cilium [43]. Rhodopsin is further transported along the ciliary plasma membrane [43]. At the base of the outer segment, flattened membrane discs are formed through evagination and subsequent expansion of the plasma membrane [43, 44]. Apparently, disc formation is a very similar process to ectosome release: it depends on actin polymerization and very likely on the ESCRT complex, ubiquitously responsible for performing scission of outward budding membranes [45]. However, in photoreceptor outer segments, the release of the formed ectosomes is inhibited by peripherin 2, making it possible to expand wide, flat membrane discs [42].
Material transfer by EVs and nanotubes
An interesting case of material transfer from one photoreceptor to others was discovered in transplant studies [46, 47]. Since the retina is part of the central nervous system, regeneration of lost photoreceptors is not happening. Therefore, many studies have examined the outcome of transplanted tissue or cells in different disease models. Although the transplants often survive for weeks, mostly detectable by the expression of fluorescent proteins like GFP, only few studies could show rare events of integration into the host tissue [48]. Revisiting those studies, it has recently been found by different groups that this integration was most often a misinterpretation of the data. Instead, material transfer seemed to have taken place from transplanted cells to host photoreceptors. At first, it was not clear, how this material transfer was happening, but secretion and subsequent uptake of free protein or free nucleic acid was excluded [46]. Secreted EVs containing GFP-positive material were found [49]. However, data suggest that random uptake of EVs was not the primary mechanism of material transfer in vivo and in vitro. Instead, a number of recent studies found that material transfer takes place through direct cell-cell connections, so called photoreceptor nanotubes [50, 51]. Nanotubes are small protrusions connecting neighboring photoreceptors [49]. The formation of nanotubes in turn depends on Rho-GTPase-dependent actin remodeling [49]. It is not yet clear, how the transfer of the material at the contact sites between nanotubes and acceptor cells ultimately takes place. One possibility could be membrane fusion permitting cytoplasmic continuity. Another solution could be the short-range release of vesicles. A combination of EV and nanotube transport could be possible, where EVs surf on the protruding nanotubes just like it has been described for exosomes surfing on filopodia [52]. Kalargyrou et al. 2021 showed that EVs derived from primary photoreceptor cultures were specifically taken up by retinal Müller cells and no other cell type, when injected into the subretinal space or vitreous of mice [51]. However, this did not hold true anymore in healthy, intact eyes without any (injection) injury, indicating that EVs are not the main route of communication between photoreceptors and Müller cells under physiological conditions. Yet, that does not exclude the possibility that Müller cells take up EVs from photoreceptors under pathological circumstances [51].
So far, nothing is known about whether and which other neurons in the retina produce EVs. Wang et al. describe that EVs are produced by rat ganglion cells in culture and taken up by ganglion cells of intravitreally injected rats [53]. However, the authors did not specify the source or isolation protocol of these ganglion cells. So, it is uncertain if cells were primary ganglion cells or of a cell line, possibly lacking some features of differentiated ganglion cells. Immunofluorescence staining of CD9 indicated localization of EVs at primary cilia of ganglion cells [30].
EVs in retinal Müller glia cells
Retinal neurons interact closely with Müller cells, radial macroglia the processes of which fill all intercellular space between the neurons. Müller cells provide metabolic support, regulate water and ion homeostasis, and modulate neuronal, microglial and vascular cell activity by releasing signaling molecules [4]. A recent review describes some so far unpublished data on MIO-M1 cells, an immortalized cell line with many Müller cell features that release EVs that contain mRNA or proteins of neurotrophic factors such as BDNF, NGF and GDNF [54]. Cultured human Müller cells released EVs with increased miR-9-3p levels under high glucose conditions, which promoted angiogenesis [55]. More in-depth analyses of Müller cell derived EVs showed that the protein content was distinctly different to that of neuronal EVs [56]. Mass spectrometry indicated that ca. 60 % of protein content in each fraction were cell type-specific. For example, the centrosomal protein 290 (CEP290) and carbonic anhydrase (CA3) were highly enriched in EVs from Müller cells. Immunohistochemical analyses indicate that EVs derived from Müller cells contained VAMP5 (myobrevin), a component of soluble N-ethylmaleimide-sensitive factor attachment proteins receptor (SNARE) complexes [56, 57]. EVs carrying components of the SNARE complex have been observed to augment synaptic vesicle fusion in target cells [57]. Immunoelectron microscopy revealed Vamp5-baring EVs in the extracellular space next to the photoreceptor side as well as at the endfeet, where the retina borders with the vitreous [56]. Strikingly, Müller glia cells released different subsets of EVs on either side of the highly polarized cells; CD81 was mainly detected in the outer plexiform layer and on apical microvilli of Müller cells, whereas CD63 expression was more prominent in the endfeet but almost absent from the apical part [56]. It is important to note that different EV populations might have distinct markers, and caution is necessary when it comes to choosing the best enrichment and characterization protocols to be able to compare EVs from different sources or even different sides of polarized cells.
Conclusions
Research on EVs in the retina has only just begun during the past few years and publication numbers are rising rapidly (see table 1 for a summary of the presence and potential functions of EVs in the context of the retina).
Table 1: Summary of the presence and potential functions of EVs in the context of the retina.
Retinal cell type | Model system | EV function / main message | Reference |
---|---|---|---|
RPE | ARPE19-cells | EVs contain Hr44 and CD63 in inflammation dependent manner | 14 |
RPE | ARPE19-cells, murine retinae, human material of AMD-patients | CD63, CD81, LAMP2 and complement factors were found on EVs; Drusen or AMD patients contained EV markers | 15 |
RPE | ARPE19-cells | Effect on immunomodulation | 16 |
RPE | ARPE19-cells | Effect on angiogenesis | 17–21 |
RPE | Polarized ARPE19-cells | Secrete EVs containing αB crystallin at the apical side | 22 |
RPE | ARPE19-cells | Inhibition of αB crystallin expression via shRNA suppressed EV secretion | 23 |
RPE | Mature, polarized primary cultures of porcine RPE cells | Differential EV cargo on apical vs. basolateral side | 24 |
RPE | Polarized primary cultures of human fetal RPE | Apical secretion of EVs containing αB crystallin | 25 |
RPE | ARPE19-cell | EVs from (H2O2) stressed ARPE19-cells, but not unstressed cells are taken up by naïve cells | 26 |
Whole retina | Murine retinal cultures | Release of EVs carrying rhodopsin | 30, 31 |
Retinal progenitor cells | Mouse retinal progenitor cells | Release EVs with enclosed miRNA, mRNA and proteins involved in multipotency and differentiation | 32 |
Rod photoreceptors | Mouse models, Xenopus laevis | Rod photoreceptors expel rhodopsin-containing EVs into the intercellular space when correct rhodopsin transport and localization to the outer segment is impaired | 33–36 |
RPE | Xenopus laevis | EVs are potentially taken up by microvilli of the RPE and degraded | 37 |
Photoreceptors | Photoreceptor degeneration mouse model | High amounts of EVs accumulate in the interphotoreceptor space | 41 |
Photoreceptors | Human embryonic kidney cells (AD-293 cells), mouse models | Photoreceptor discs form through peripherin-dependent suppression of ciliary ectosome release | 42 |
Photoreceptors | Review on different models | Photoreceptors are built like ectosomes | 45 |
Photoreceptors, Müller cells | Mouse models, primary photoreceptors, retinal cultures and co-cultures | Müller cells take up EVs derived from primary photoreceptor cultures when injected into subretinal space | 51 |
Ganglion cells | Retinal ganglion cell culture, rat | EVs are produced by rat ganglion cells in culture and taken up by ganglion cells of intravitreally injected rats | 53 |
Müller cells | MIO-M1 cells | EVs contain mRNA or proteins of neurotrophic factors such as BDNF, NGF and GDNF | 54 |
Müller cells | EVs from vitreous humor of proliferative diabetic retinopathy patients, hRECs (human retinal microvascular endothelial cells), hMGs (human Müller glia cells), hMC3 (human micro glia cell line), ARPE19-cells | hMGs released EVs with increased miR-9-3p levels under high glucose conditions, promoting angiogenesis | 55 |
Müller cells, retinal neurons | Mouse, murine retina cultures | Protein content of Müller cell derived EVs was different to that of neuronal EVs; EVs derived from Müller cells contained VAMP5; Müller glia cells released different subsets of EVs on either side of the highly polarized cells | 56 |
Many studies focus on the therapeutic potential or on EVs as biomarkers for retinal diseases. Yet, rather little is known about the physiological release and uptake sides, let alone function. Most research was performed on cell cultures. However, not many groups have performed thorough characterization of EV samples. Future studies on EVs in the intact retina under healthy and pathological conditions are necessary, and the identification of content and its function should be prioritized. Intercellular communication between various retinal cell-types is mandatory to maintain a functional homeostasis. So, not only the differences between released EVs from distinct cell types are necessary, but also their interplay should be addressed by co-culture experiments and in vivo studies. On top, it would be very interesting to find out, whether and how light conditions, circadian rhythm and developmental stage alter the production, content and release of EVs in the retina. There is still a lot more to elucidate about retinal EVs in the future!